NTNU Nano
For updated information regarding NTNU NanoLab's activities, please go to NanoLab's homepage.
NTNU Nano
The study, manipulation and exploitation of nanostructured materials and devices lies at the heart of modern science and technology. NTNU – the Norwegian University of Science and Technology – is home to the largest centre for nanoscience and nanotechnology in Norway. Read on to discover some of the ways in which nano-related education and research at NTNU is creating knowledge for a better world.
News 2023
Materials scientists who work with nano-sized components have developed ways of working with their vanishingly small materials. But what if you could get your components to assemble themselves into different structures without actually handling them at all?
Read more about how Verner Håkonsenooks up tiny supercrystals in NTNU NanoLab here.
“Conductive tests across FIB-welded aluminium-copper interfaces” by Ambra Celotto
An effective technique to obtain sound joints of ductile materials is to apply pressure that imparts plastic deformation for bringing virgin surfaces into contact at the atomic scale. The bonding mechanism between two dissimilar metals typically involves atoms diffusion, which is facilitated by high temperatures. But what if we carry out the process at the room temperature? It seems that enough plastic deformation alone is also able of inducing atoms diffusion! Therefore, for investigating this phenomenon in situ, a downscaled version of the conventional Cold Pressure Welding technique was developed inside the FIB. This consists in a tailored configuration of the lift-out needle employed to squeeze a small copper sample within aluminium walls. Quite an unusual application for the Focused Ion Beam.
The obtained joint was then milled into three bars at different depth to perform conductivity test across the bonded interfaces. By running current through miBots™ manipulators, it is possible to assess whether the electrical conductivity of the base metals is altered by the joint and thus benchmark the welding technique against the conventional ones for electronic applications.
Researchers at NTNU are searching for materials that could be used to make Li-ion batteries with a higher energy density.
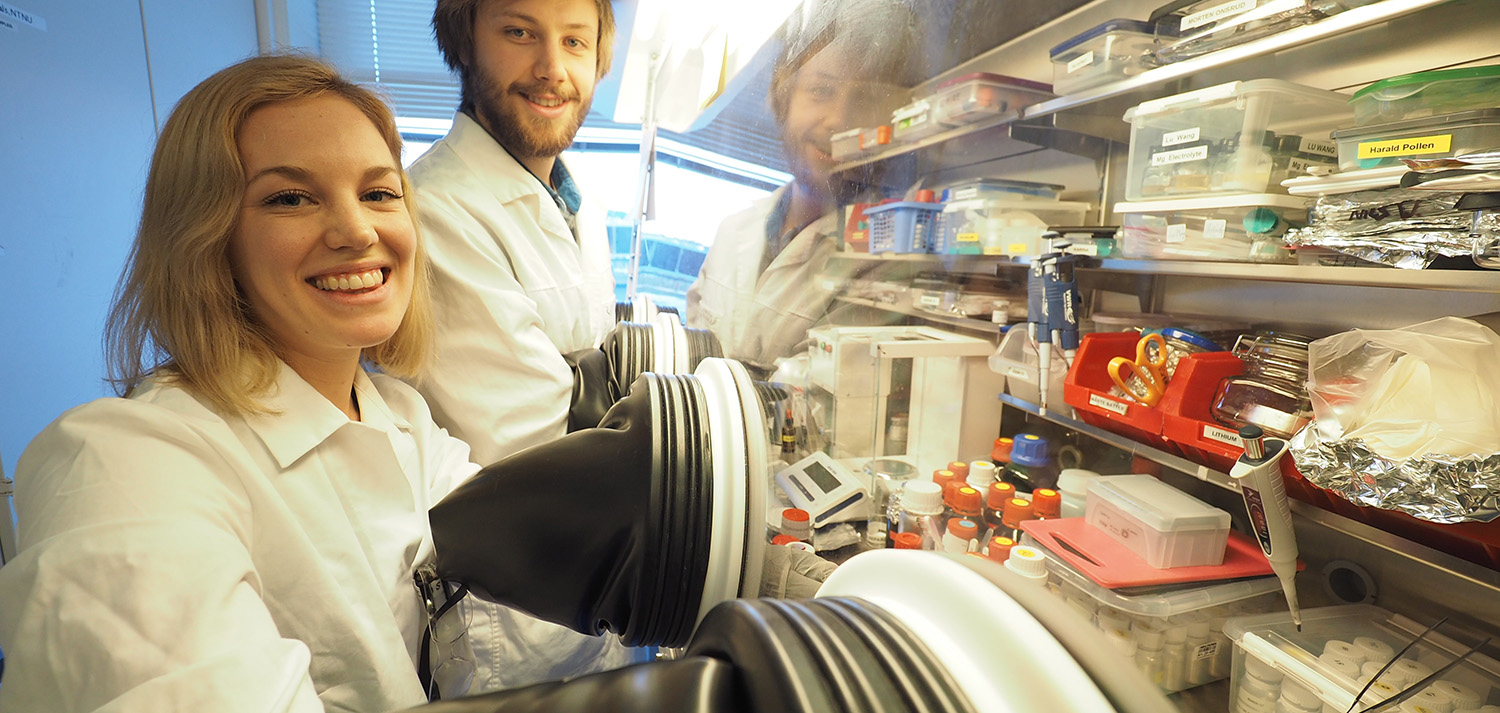
From smartphones to electric cars, lithium ion batteries have changed the way we power our lives. And in the push towards net zero carbon emissions globally, they will be a vital part of decarbonising transport networks and switching to renewable energy.
To make batteries with a higher energy density – which would mean that an electric car, for example, could cover more distance before it needed recharging – researchers at NTNU are investigating the use of different materials for the battery’s key components.
Swapping silicon for graphite
Batteries store energy by moving charged particles between two electrodes – a negatively charged anode and a positive charged cathode – through a liquid known as an electrolyte. In lithium-ion (Li-ion) batteries, lithium ions move from the cathode to the anode when the battery is charged, and are stored in the anode. When the battery is discharged, the ions move back to the cathode, producing an electric current.
Most existing Li-ion batteries use graphite for their anode. But swapping graphite for silicon could increase the energy density significantly. “In lithium ion batteries, you would like to have anode and cathode materials that can store as much lithium as possible,” says Ann Mari Svensson, a professor in NTNU’s Department of Materials Science and Engineering. “Silicon can store huge amounts.”
Combatting flammability
But Li-ion batteries have a reputation for being flammable. Switching out the electrolyte – the crucial component through which ions move between electrodes – is the main way to combat this.
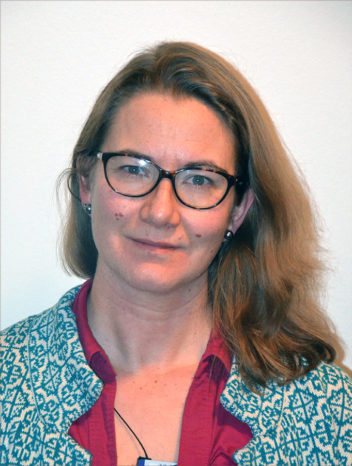
Photo: NTNU
Svensson and colleagues are investigating the use of ionic liquids – essentially, salts that have such a low melting point that they are liquid at room temperature – to make batteries with a silicon electrode safer. “The current liquid electrolytes are partly to blame for thermal events,” says Svensson. “If you can replace them with, for example, new ionic liquids, then the battery is less likely to catch fire.”
In work published in the Journal of The Electrochemical Society and funded by the Research Council of Norway, Svensson and colleagues evaluated the performance of batteries with silicone anodes and ionic liquid electrolytes at high temperatures.
Increasing battery temperature helps
Increasing the temperature of the battery increases the conductivity of lithium ions within the electrolyte, overcoming the fact that ionic liquids don’t tend to conduct lithium ions very well at room temperature. Demonstrating that these batteries can work at elevated temperatures could also make operating large packs of batteries, that have a tendency to overheat, simpler. “Normally you have to cool the pack when it's operating. That could be, in theory, avoided,” says Svensson.
During the course of their research, the team saw some surprising results. “We had studied these ionic liquids at room temperature before, but when we heated them to sixty degrees, then actually, the best one wasn't the best one anymore,” says Svensson. “The ranking was quite different.”
“One of them had a really excellent improvement in conductivity when going to the higher temperatures,” she added.
Thin film on silicon is key
One driving factor behind some ionic liquid electrolytes performing better than others could lie in a key feature of Li-ion batteries with silicon electrodes: a thin film that forms over the silicon. When you start to charge a battery, lithium moves from the cathode to the anode. But in the process, the electrolyte starts to decompose and forms a thin film called a passivation layer on the surface of the anode. “Once they're really covering the surface, the formation of these layers stops,” says Svensson. “It's very important that these are intact, because otherwise you would just completely decompose most of your electrolyte.”
For the ionic liquid electrolytes that performed well at the higher temperature, the researchers saw evidence of a stronger passivation layer as well as improved lithium ion mobility in the electrolyte.
More work before commercialisation
Svensson and colleagues use something called X-ray photoelectron spectroscopy, or XPS, at NTNU’s NanoLab, to investigate the films. “You need quite some advanced equipment to try to identify those films, they can be just a few nanometers thick,” says Svensson.
After showing that ionic liquids can make a silicon anode a realistic option for Li-ion batteries, Svensson and her colleagues are now working on demonstrating how an ionic liquid electrolyte would work with a new type of cathode, too.
There are still many hurdles to overcome before these kinds of batteries could be available commercially. For one, ionic liquids are not currently mass produced in quantities that would be required for industrial production of batteries using them. And while research has shown promise when it comes to their short term performance, their long term performance – over the expected lifetime of a battery in the real world – remains to be seen. “That's quite a big challenge,” says Svensson.
Kelly Oakes, September 2023
Reference: Daniel Tevik Rogstad et al 2022 J. Electrochem. Soc. 169 110531
DOI: 10.1149/1945-7111/ac9f78
Funding: This work was performed within MoZEES, a Norwegian Centre for Environment-friendly Energy Research (FME), co-sponsored by the Research Council of Norway (project number 257653) and 40 partners from research, industry and public sector. Institute for Energy Technology (IFE) is acknowledged for providing the Si electrodes. The Research Council of Norway is acknowledged for the support to the Norwegian Micro- and Nano-Fabrication Facility, NorFab, project number 245963/F50.
Onsager Professor Maria Helena Godinho, visiting from New University of Lisbon, Portugal, presented her Onsager lecture at NTNU on August 24th.
The lecture, entitled "Self-organized cellulose-based liquid crystalline systems: a world of possibilities", covered the wondrous world of cellulose.
Her research includes the possibility of extracting colour from cellulose, which is a carbohydrate found in the cell wall of plants. It provides the plant with strength, helps the plant to grow upright, and is also important in the cell division of plants. It is the most abundant organic polymer on Earth. The cellulose content of cotton fiber is 90% and that of wood is 40–50%.
Per Henning August 2023
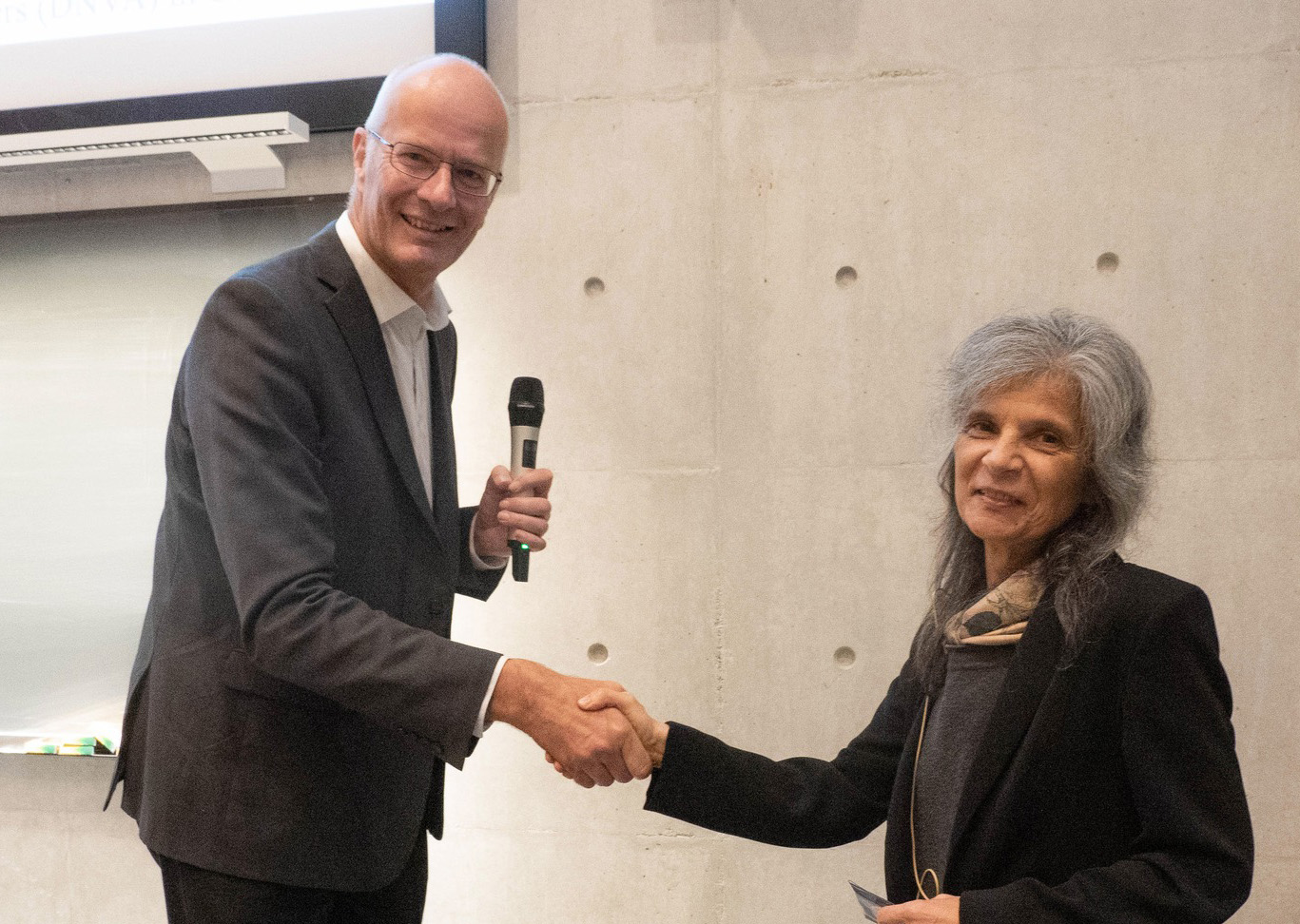
Researchers at NTNU have found a way to make gold nanoparticles with a uniform size and shape, opening up the possibility of finding more effective photocatalysts.
When organic pollutants such as dyes, agricultural chemicals, and pharmaceuticals enter waterways all around the world, they can harm the environment and human health – and removing them can be incredibly difficult.
Photocatalysts – substances that absorb energy from light and use it to accelerate the rate of a chemical reaction – can decompose organic pollutants in a process called mineralisation, converting them into water, carbon dioxide, and other harmless molecules. But there’s a catch: most photocatalysts require UV light to work, making them impractical and expensive to use at scale.
To solve that problem, researchers have their sights set on finding a photocatalyst that can harness much more of the solar spectrum. “If you can use solar light, it's cheaper and much more available than UV light,” says Magnus Rønning, a professor in catalysis at the Department of Chemical Engineering, NTNU.
Gold nanoparticles key
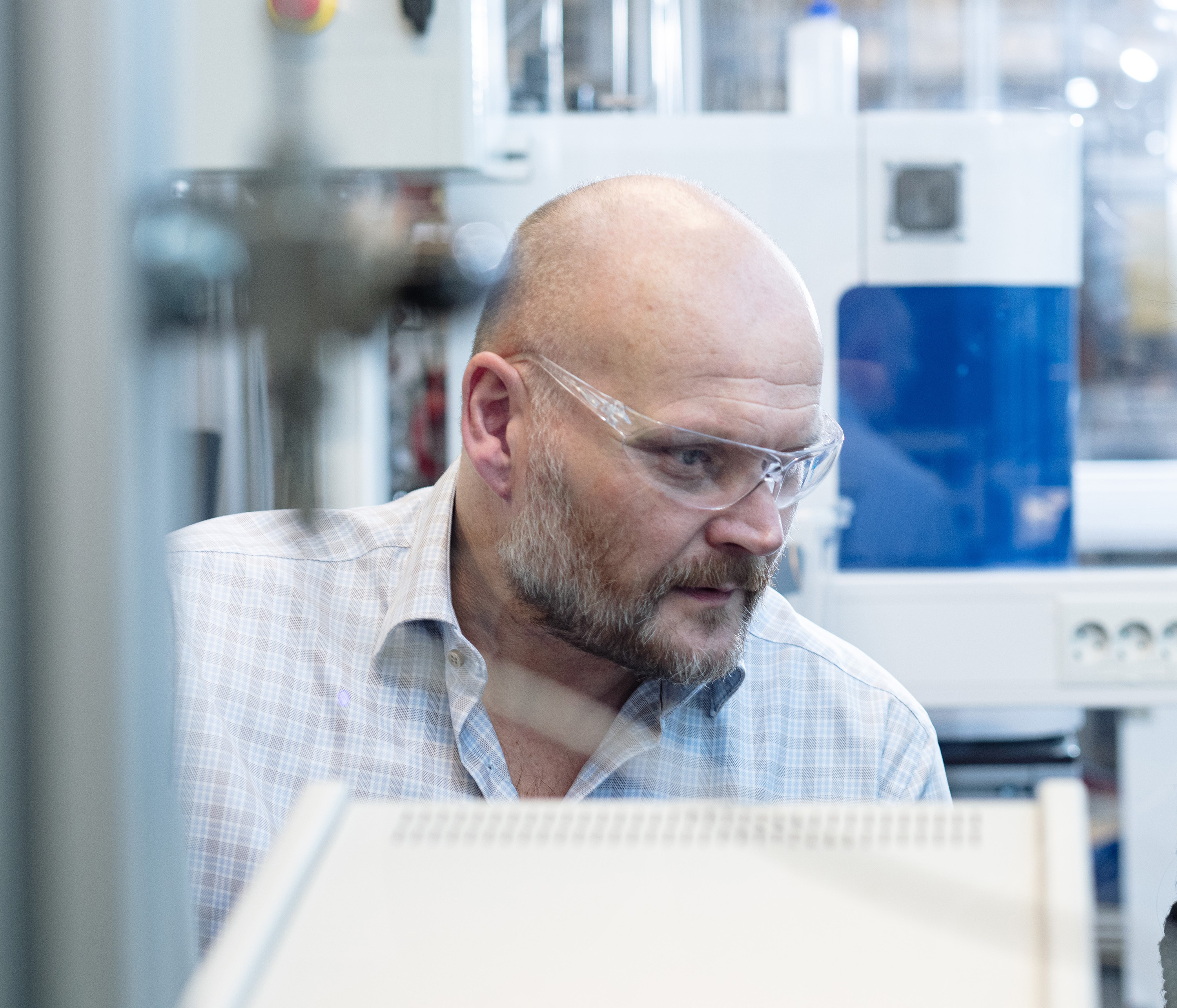
Photo: Kim Ramberghaug
Nano-sized disks made of the mineral bismutite are a promising photocatalyst, and researchers have discovered that adding gold nanoparticles to their surface increases their sensitivity to the visible part of the solar spectrum. However, while there are several ways to deposit those gold nanoparticles on a surface, most methods give limited control over the size and shape of the particles you end up with. “Often you will get a distribution of sizes and shapes [that] you cannot really control, so you have a mix of rods and spheres and cubes,” says Rønning.
Now, Rønning and colleagues at NTNU have found a way to create gold nanoparticles with a uniform size and shape on the surface of the bismutite nanodisks. Their research, recently published in the journal Photochemical and Photobiological Sciences and part funded by the Research Council of Norway, opens up the possibility of studying the effect of both the size and shape of the nanoparticles on the performance of the catalyst, in turn making it possible to maximise its light-harvesting capacity.
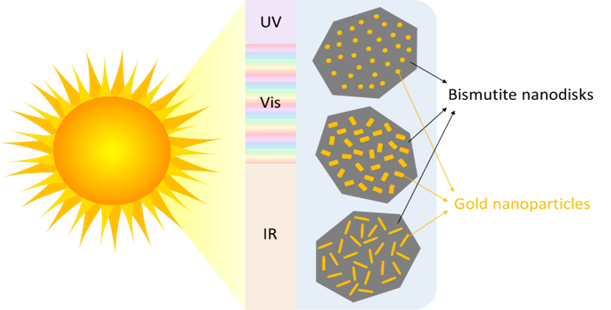
Tested different shaped nanoparticles
The researchers used small gold seeds as nucleation sites on which they grew gold nanoparticles in different shapes – rods, etched rods with roughened surfaces, and spheres – by adjusting the concentration and pH of the solution the particles were growing in.
These nanoparticles have a surfactant layer on their surface, which reduces the likelihood they will clump together, before they are deposited on the bismutite nanodisks
“With this, we can keep a reasonably good control over the size and shape of these particles,” says Rønning. The samples were prepared and characterised by PhD candidate Jibin Antony in NTNU’s NanoLab, with the catalytic reactions themselves run in the labs of the Catalysis Group in the Department of Chemical Engineering.
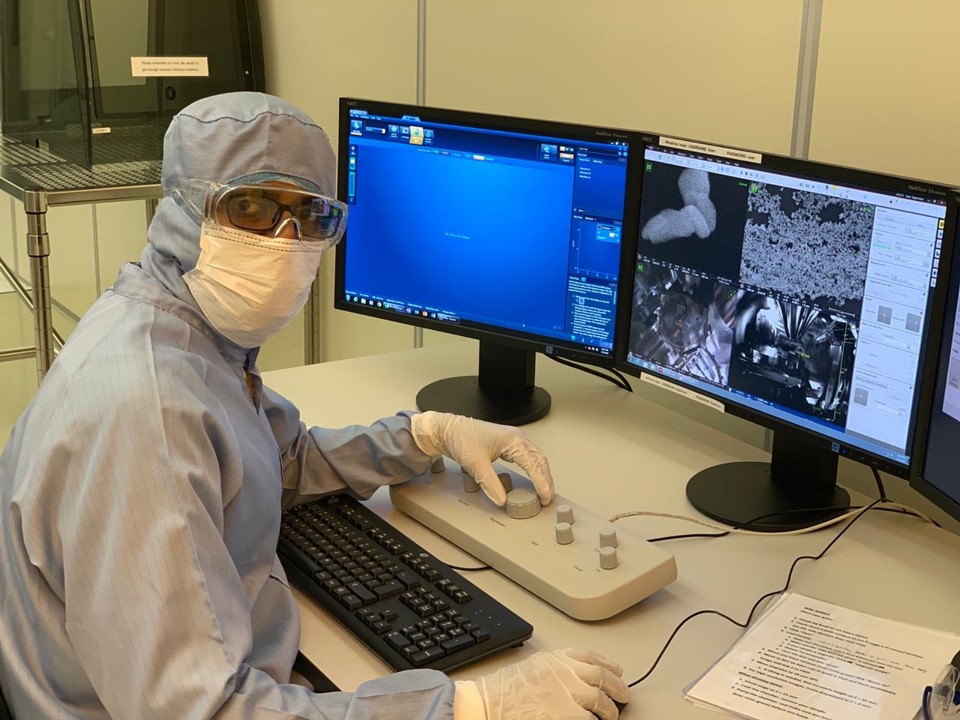
Promising tests
The researchers tested how well the resulting photocatalyst could break down an organic pollutant known as methylene blue. As well as being a widespread organic contaminant in its own right, methylene blue is a useful test case for how well a photocatalyst will work on other pollutants. “It's quite representative as an organic contaminant, but it's also a relatively complex molecule,” says Rønning. “If it works on methylene blue, it should also work well on other organics.”
The other advantage of using methylene blue is that its decomposition is already well understood, allowing the researchers to probe not just how much methylene blue is left at the end of the process, but what it’s been converted to. While that is not something that Rønning and colleagues looked at in their work on gold nanoparticles, in a related paper the researchers saw that adding silica to bismutite nanodisks did change the degradation products of methylene blue. “In the end, you want full mineralization and not just conversion into something that is just as dangerous or unwanted as the methylene blue,” says Rønning.
Rods better than spheres
Rønning and his colleagues found that the photocatalyst with rod-shaped gold nanoparticles performed 14% better than the one with spheres. But there is still room for improvement. “Even after three hours of reaction, you still have some of the contaminant left,” says Rønning. “So, yes, it's working. But we still need something that works better.”
Some photocatalysts are already used in wastewater treatment and air purification systems commercially. The technology also holds promise for hydrogen splitting – producing cheap hydrogen fuel using just water and sunlight. However, to make that possible, researchers need to find a way to make the catalysts much more effective than they are today.
The key improvement needed for better photocatalysts rests on how many of the photons are actually used to drive the reaction. “In good cases, it's maybe 1%,” says Rønning. “If we can get this up to, say, 10%, it will be much closer to practical applications.”
While changing the size and shape of gold nanoparticles is unlikely to bring about such a huge increase in efficiency, it's a start. “We need to improve the catalyst in order for this to be commercially viable,” says Rønning. “This is a step in that direction.”
Kelly Oakes, June 2023
Reference: Antony, J., Bandyopadhyay, S., Yang, J. et al. Optimizing the shape anisotropy of gold nanoparticles for enhanced light harvesting and photocatalytic applications. Photochem Photobiol Sci (2022). https://doi.org/10.1007/s43630-022-00351-8
Funding: The Research Council of Norway is acknowledged for the support to the Norwegian Micro- and Nano-Fabrication Facility, NorFab, project number 295864.
Norway has seen an increase in solar power capacity in recent years, but in winter solar panels face a big problem: snow. Researchers at NTNU modelled how much extra electricity could be generated if solar panel surfaces were designed to repel snow and ice.
Norway has been slow to exploit solar energy, but over the last few years interest in the technology has been rapidly increasing: between 2015 and 2021, the country saw a 15-fold increase in its capacity for solar power generation.
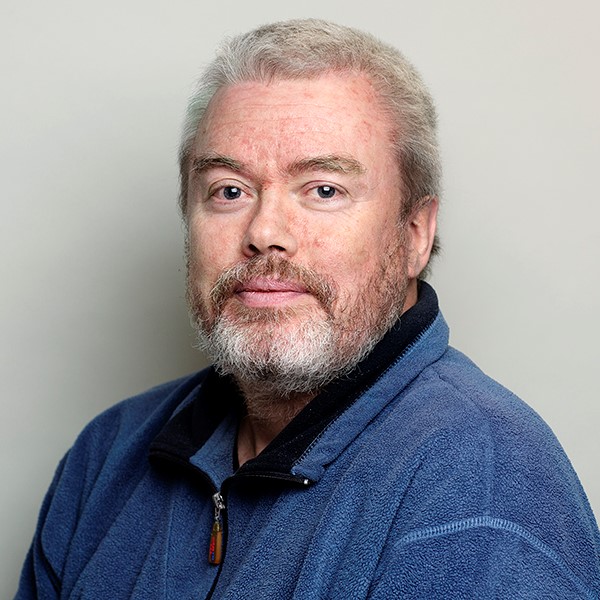
“It's a classical misunderstanding that there is not enough solar radiation in Norway,” says Professor Bjørn Petter Jelle at Department of Civil and Environmental Engineering at NTNU. “In one way, it’s partly true: we don’t have so much sun in the darkest winter months. But on the other hand, the sun we do have in winter is very valuable. In addition there is, of course, midnight sun or sunshine large parts of the night during summer.”
There is one significant challenge to overcome, however: snow on solar cells can reduce the amount of electricity they generate at the time of year when it is needed the most. “A thin layer of snow and you have no electricity production,” says Jelle.
Complex problem
Preventing snow and ice from sticking to a solar panel is a more complex problem than it might first appear. Depending on many variables and their interactions, snow may at times easily slide off and at other times stick to the solar cell panel surfaces.

Photo: Clara Good
To understand the impact of the snow problem on Norway’s solar power generation, Jelle and colleagues at NTNU modelled how much extra electricity could be generated in three Norwegian cities if solar cells featured icephobic surfaces or coatings that reduced the amount of snow accumulating on panels.
Simulated icephobic surface
Using NTNU’s ZEB Living Lab in Trondheim as their case study, Jelle and colleagues estimated how much solar radiation would be reaching the surface of the building’s solar cells throughout the winter months – and how much solar electricity would have been lost due to snow coverage over the last four years. Then, using data on the efficiency of icephobic coatings available commercially for other applications, the researchers simulated how much of the electricity lost due to snow coverage could be recovered if those solar panels had had an icephobic surface. The researchers then repeated the same analysis for two other cities, Oslo and Bergen, using local climate and latitude data.
They found that icephobic coatings could reduce the amount of electricity lost over the winter months by 65% in Oslo, 60% in Trondheim, and 45% in Bergen. The work was funded by the Research Council of Norway and published in the journal Solar Energy.
November and December less important
The research also showed that the coatings would be most effective between January and April, rather than November and December, at all three locations. This makes sense when you consider the difference in the amount of solar radiation over those months, says Jelle. In Trondheim, the city at the highest latitude in the study, there are approximately four to five hours of daylight during the dark months of December and January. “Then as you get into February and March, you get more and more sun,” says Jelle. That extra solar radiation would also start to melt snow that’s accumulated on a solar panel, thus giving an icephobic coating a helping hand.
As yet, though, the icephobic coatings simulated in the study are not yet available commercially for solar cells. Those designed for other applications, such as coatings based on silicones used on aircraft, tend to be opaque – something that would defeat the purpose of using it on a solar panel. “If you add a coating on the surface for a purpose other than trapping solar radiation, then you're often reducing the solar radiation harvesting,” says Jelle.
However, researchers are currently developing nanostructured surfaces that could repel ice and snow without the need for an additional coating. Often, these are designed so that any water hitting the surface is repelled before it freezes, preventing the formation of a layer of ice that snow can more easily stick to. But whether it's possible to create a surface that fully prevents ice or snow accumulation is still an open question. “It is possible to delay it, but if you can delay it and then at the same time manage to keep the snow off for a long time, that's much more difficult,” says Jelle. “Even if the surface is very smooth, snow can still stick.”
If researchers do manage to create such a surface, it would be useful across many industries. Given the right conditions, snow and ice can accumulate on airborne power lines, aircraft hulls and wings, ship hulls, road signs and a lot of other surfaces, causing a whole host of problems. “Driving a car in Norway in winter, often you cannot see what's on the signs because they're covered by snow or ice,” says Jelle.
Reference: Mattia Manni, Maria Chiara Failla, Alessandro Nocente, Gabriele Lobaccaro, Bjørn Petter Jelle. The influence of icephobic nanomaterial coatings on solar cell panels at high latitudes, Solar Energy, 248, 76-87 (2022). https://doi.org/10.1016/j.solener.2022.11.005
Grant acknowledgements: Research Council of Norway projects “Enhancing Optimal Exploitation of Solar Energy in Nordic Cities through the Digitalization of the Built Environment” (Helios, project no. 324243), “Building Integrated Photovoltaics for Norway” (BIPV Norway, project no. 244031) and NorFab (National infrastructure, project no. 295864.
Kelly Oakes, April 2023
By using a cutting edge technique to observe what’s happening at the atomic level inside their material, researchers at NTNU have discovered a surprising new method to make aluminium alloys stronger.
Humans have been mixing metals to create more useful materials for thousands of years. The Bronze Age, which started around 3300 BC, was characterised by the use of bronze, an alloy of copper and tin which is stronger than either metal alone. Now, researchers at NTNU have discovered a counterintuitive way to make a much more recent invention – nanograined alloys, featuring nano-sized grains of the alloying element – even stronger.
Aluminium is a metal that is widely used to make components in the aerospace, transport, and construction industries, in part because it is lightweight yet durable. Alloys of aluminium retain these qualities but are stronger than aluminium alone. “If it was a pure aluminium, of course, it's not strong enough,” says Yanjun Li, Professor of Physical Metallurgy in the Department of Materials Science and Engineering at NTNU.
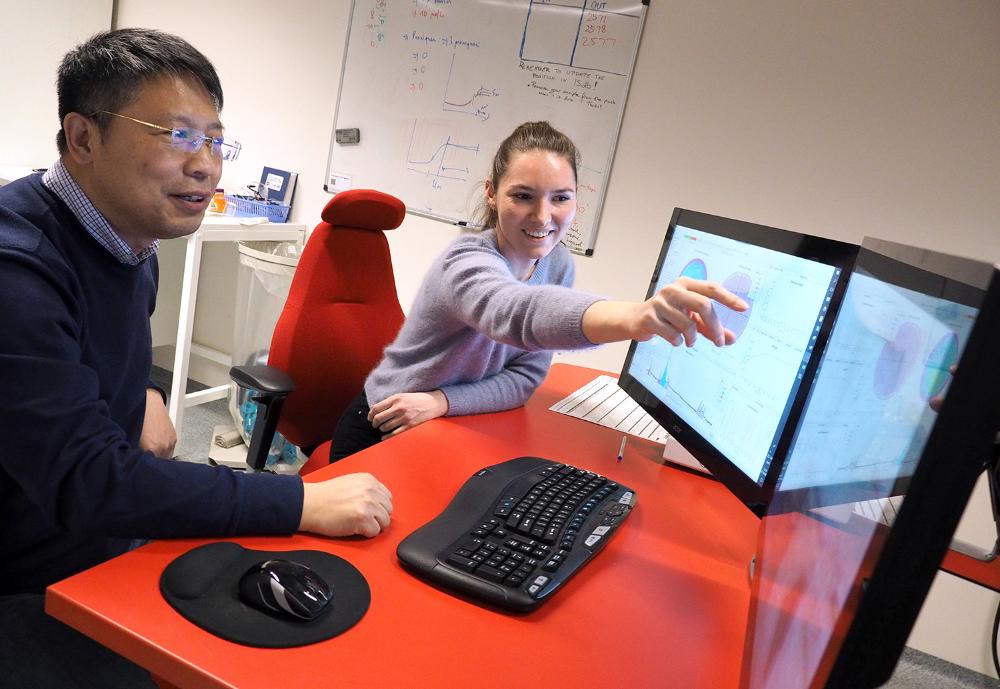
Large particles decrease strength
But in recent years, researchers attempting to make nanograined alloys of aluminium containing copper have run into a problem: the copper atoms have a tendency to clump together, forming coarse particles with aluminium inside the material, especially at temperatures higher than 100 oC. When the copper is no longer evenly distributed throughout the material, the alloy becomes weaker. “They accumulate together, forming large particles,” says Li. “These particles, when they are large, can actually decrease the strength.”
Adding vacancies for stability
Copper atoms can move through material if there is a so-called vacancy – a space not occupied by atoms – that they can move into. So researchers have been trying to minimise the number of vacancies to reduce the ability of atoms to move. “If there are no vacancies, of course no atoms could move,” says Li.
But now Li and his colleagues have found a way to increase the number of vacancies and at the same time increase the strength of the resulting alloy.
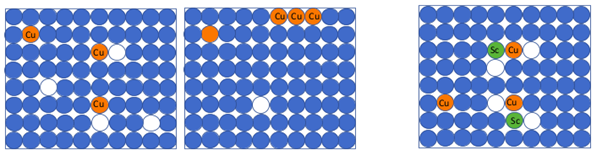
Copper atoms alone can easily move through the material by exchanging positions with vacancies as shown in (a), as a consequence they can easily clump together forming large Al-Cu intermetallic particles (b), but when combined with scandium atoms and vacancies they form clusters that remain more stable (c). Graphic: Yanjun Li / NTNU
In work partly funded by the Research Council of Norway and published in the journal Nature Communications, the researchers added scandium atoms as well as copper ones to aluminium, while also increasing the number of vacancies. The scandium and copper atoms, together with the vacancies, formed structures that could not easily move through the material. “Together they are very stable,” says Li. “It becomes more difficult for any of them to move.” Thanks to the new scandium-copper structures, large aluminium-copper particles that would have previously formed were completely suppressed, even when the alloy was heated to 200 oC for 24 hours. This stability means the copper atoms stay evenly distributed throughout the material, and the alloy retains its added strength.
Atom probe tomography key
The team saw the copper-scandium-vacancy clusters using Atom Probe Tomography (APT), a technique which makes it possible to see what’s happening at the atomic level inside a material. PhD student Hanne Søreide prepared very thin needles – only 50 nm in diameter – of the alloy using NTNU Nanolab’s Focussed Ion Beam. She then used the atom probe to evaporate atoms, one by one, from the top of the needle, while a detector captured information about them. “Different atoms can fly faster or slower,” says Li.
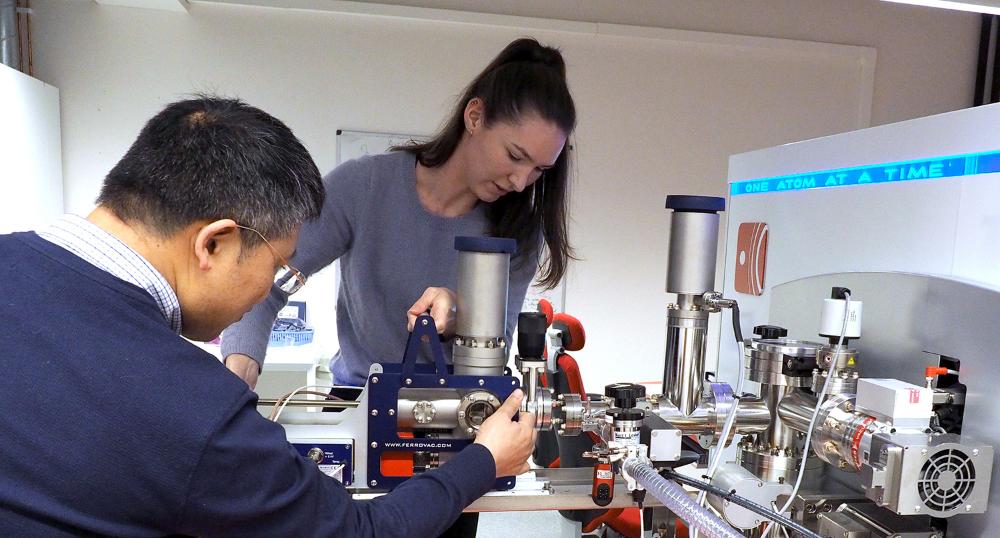
Using this information, the researchers reconstructed a picture of where each atom originally was in the material. They saw that atoms of the two different alloying elements were joining together inside the aluminium. “They are bonding together, we can detect this with copper and scandium,” says Li. “They are really closely connecting together.” The researchers confirmed their findings using transition electron microscopy.
50 per cent stronger
Li says the team have already used these findings to make an aluminium alloy that is 50% stronger than alloys containing the same amount of copper that are currently available commercially. “This is not an easy task for aluminium,” he says. While the materials Li and colleagues are making in the lab are still far from application in industry, alloys that retain their strength at high temperatures are useful in engines and for other applications where the components get hot.
NTNU’s atom probe lab is the first facility of its kind in Norway. Li and his colleagues hope that using APT will help them find other new materials with desirable properties. “By really understanding the material in the atomic scale, it can help us to design new alloys, new materials with even higher strength,” says Li. “Without this kind of instrument, it's almost impossible to understand these materials.”
Kelly Oakes, February 2023
Reference:
Wu, S., Soreide, H.S., Chen, B. et al. Freezing solute atoms in nanograined aluminum alloys via high-density vacancies. Nat Commun 13, 3495 (2022). https://doi.org/10.1038/s41467-022-31222-6
Grant acknowledgements:
The Research Council of Norway supports the Norwegian Laboratory for Mineral and Materials Characterisation, MiMaC, project number 269842.
News 2022
At this inspiring international meeting, scientists are invited to discuss in a relaxed atmosphere recent advances in understanding the interaction of light with nano and microscale systems. The meeting will have a strong emphasis on encouraging interactions between senior and early stage scientists, with technical and social activities all day long, from breakfast until after dinner.
Updated information about the program, the venue, fees and registration may be found on the DINAMO homepage.
The registration is open until Feb 1st, 2023.
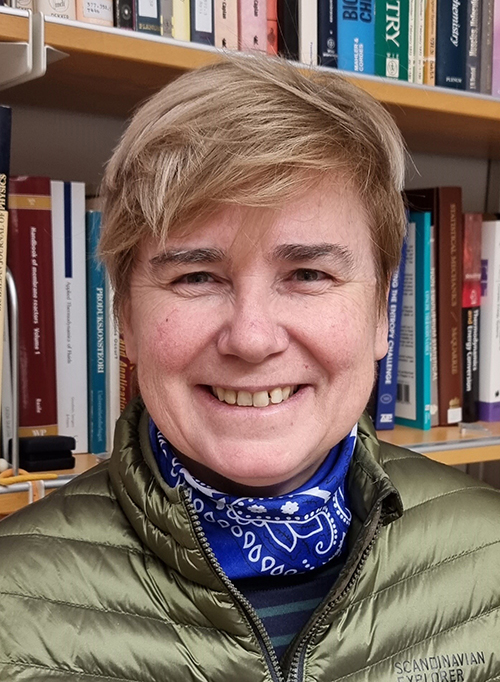
Erika Eiser is a Professor in NTNU’s Department of Physics and a Principal Investigator at Porelab. Here, she speaks with NTNU Nano science writer Kelly Oakes about her current research and plans for the future.
We are happy to announce that newly established research school in micro- and nanotechnology opens registration for PhD candidates, post doctoral researchers and their supervisors.
Research School for Training the Next Generation of Micro- and Nanotechnology Researchers in Norway (TNNN) is supported by the Research Council of Norway and will establish a vibrant national network of junior scientist working in Micro- and Nanotechnology. It will provide training in transferable skills and facilitate collaboration with industry.
More information and registration: https://www.ntnu.edu/tnnn/tnnn
We are pleased to announce a new scheme for funding inward research visits to three of NTNU's leading nanoscience infrastructures: NTNU NanoLab, NORTEM and MiMac. The scheme will provide funds of up to ~200 kNOK for researchers from leading international research groups to carry out innovative, collaborative research projects at the three infrastructures. Projects must have an NTNU-based collaborator and should be completed before July 2023. Proposals should be submitted by the NTNU collaborator by Sep. 1 2022, using this online application form.
Full application details may be found here.
Best wishes
John de Mello
A list of NTNU papers related to nanoscience, nanotechnology and functional materials published in March - May 2022 may now found on NTNU Nano's page for publications.
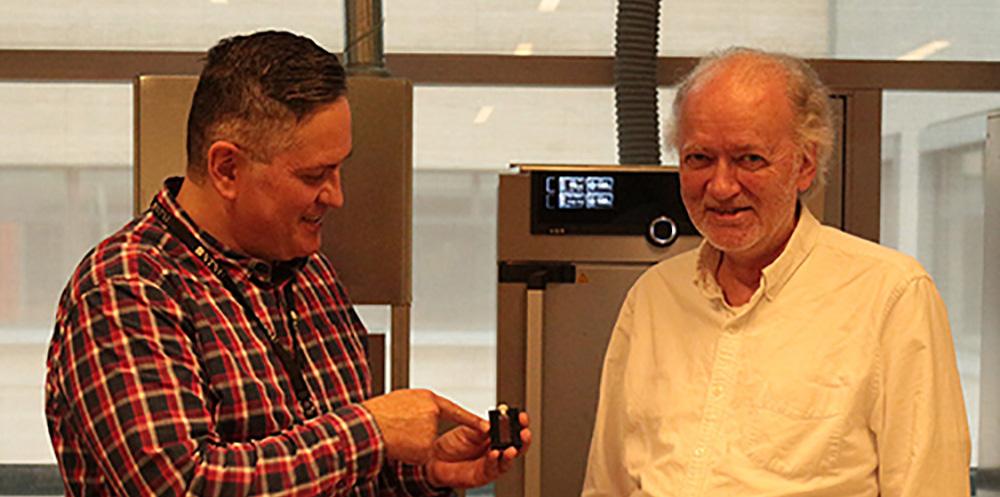
Birds, butterflies, and many other natural objects get their bright colours from intricate structures on their surfaces. Now researchers at NTNU have used the same principle to create colours with clay nanostructures
When choosing paint for a wall, or tiles to decorate a bathroom, we might take inspiration from nature. But there’s a good reason why, right now, our walls don’t truly live up to the bright blue of a peacock’s feather or the deep orange of a butterfly’s wing.
Colours can be produced in two fundamentally different ways: chemically or physically. Our existing paints, plastics and cosmetics contain chemical pigments that absorb all wavelengths of light except for one, which is reflected, giving them a corresponding colour. Physical or structural colours, on the other hand, are produced not by light-absorbing chemical pigments but by the way the light interacts with a nano-sized structure. “This is what we find in nature,” says Jon Otto Fossum, a professor in the Soft and Complex Matter Lab at the Department of Physics, NTNU.
The nanostructures themselves – found on a bird’s feather, the wing of a butterfly, or even the berries of some plants – are almost transparent. “You shine white light on it, and what actually happens is that most of the light goes straight through, but some light is reflected from each interior interface,” says Fossum. It is the interaction between these reflected rays, known as constructive interference, that creates the colour. Rainbows and soap bubbles get their colours the same way.
Mimicking soap bubbles
Now, in work funded by NTNU and by the Research Council of Norway, Prof. Fossum, together with other researchers at NTNU and at the University of Bayreuth in Germany, have found a way to create structural colours using very thin sheets of clay suspended in water. If these clay suspensions can be solidified and then ground into pigments, they could replace the toxic chemical pigments currently used in paints, textiles, and even cosmetics, creating long-lasting colours that don’t fade over time.
There are two different approaches to making structural colours in the lab. Some researchers try to mimic structures found in nature. Lexus, for example, sells cars in a structural blue that mimics a butterfly’s wing. “Those are very intricate structures,” says Fossum. “It's not a simple stacking of sheets, it’s like a small Christmas tree on the nanoscale.”
But that is a laborious and time-consuming task – Lexus says it takes eight months to produce enough structural blue pigments for 300 cars. With their clay structures, Fossum and his colleagues have used the same underlying principle but implemented it in a simpler way. “They don’t mimic the exact structure, they mimic the principle,” he says. “It’s more like mimicking soap bubbles than mimicking birds.”
Self-assembly
The clay structures, made by NTNU postdoctoral researcher Paulo Michels-Brito, are formed in distinct self-assembly steps. The clay arrives from the University of Bayreuth as a powder, where the powder grains are nano-sized stacks with sodium ions in between each clay layer.
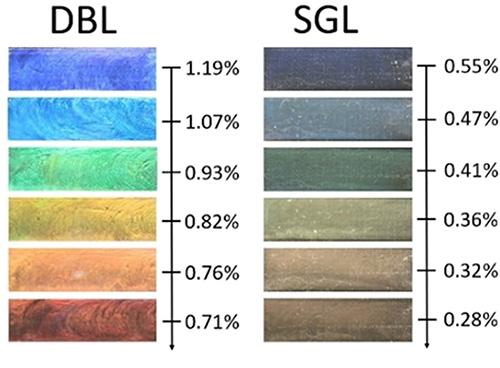
brighter colours than the mediocre colours produced
by single-layered (SGL) sheets, and the colour they
produce varies with the concentration of clay
(given in volume %). (Fossum et al 2022)
First the clay powder is suspended in water containing caesium salt, and caesium ions insert themselves in between every second layer, with sodium ions remaining in the other interlayers. In a second spontaneous process, the clay stacks are “cleaved” apart at the sodium interlayers, producing a suspension of double layered nanosheets. Finally, in a third spontaneous self-assembly process, the distances between the double-layered nanosheets are tuned either by varying the clay concentration or by varying the amount of salt in the water. The researchers initially used NTNU’s NanoLab to study the fundamental properties of the clays used for creating the nanostructures.
The idea to make each sheet a double layer came during discussions with collaborators at the University of Bayreuth. The double layers are less transparent than the single clay nanolayers, so they produce much brighter colours than in the single clay layer case. “The most important hurdle that we already passed is to make the double layers,” says Fossum. “That is not straightforward.”
Another hurdle that we have overcome with our way of making structural colours is that we can produce structural red as easily as can we produce the whole visible spectrum. It has been well explained and demonstrated by several groups worldwide why structural red production is notoriously difficult both in natural cases and by using artificial nanostructures. Our solution to the “red problem” is still another factor that makes our concept interesting for industrial upscaling.
As in nature, the separation between one double layer and another is what determines the colour the clay produces. “What happens with the chameleon is that it stretches the skin and changes distances, and that changes its colour,” says Fossum. Chameleons also have dark skin below their colour producing nanostructure to absorb the light that passes through the structure, a strategy Fossum and colleagues also adopted.
Many structural colours found in nature are iridescent, meaning they change when viewed from a different angle. But having a colour that is fixed no matter the viewing angle is more useful for many human applications – and it requires a relatively small amount of disorder in the structure. “We get that automatically in our system because the clay platelets buckle, they are not stiff,” says Fossum.
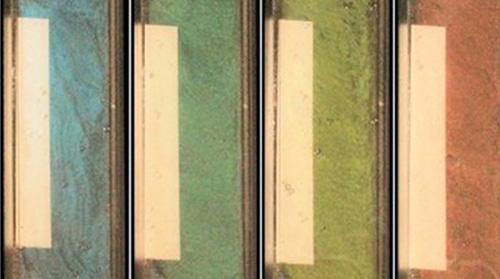
Next steps
Having made structural colours with synthetic clays, the researchers are now extending this concept to natural clays, which come with more defects and impurities that make the process trickier. But natural clays have the advantage of being widely available. “If you want to, let's say, paint all the houses in the world with such new structural pigments, we have to go over to natural clays,” says Fossum.
As well as paints, the clay structures could be added to textiles and cosmetics, says Fossum. But first the researchers need to find a solid, transparent carrier matrix to replace the water, so that the distances between the double layers are maintained when the structures are ground into pigments. That matrix will make up the vast majority of the material, so getting it right is vital. “That's why we’d like to develop a matrix that is a recyclable, sustainable material, so they don't contribute to more microplastics in the oceans,” he says.
Kelly Oakes, May 2022
Reference:
Bright, noniridescent structural coloration from clay mineral nanosheet suspensions, Paulo H. Michels-Brito, Volodymyr Dudko, Daniel Wagner, Paul Markus, Georg Papastavrou, Leander Michels, Josef Breu, Jon Otto Fossum, Science Advances 8(4), DOI: 10.1126/sciadv.abl8147 (2022)
Grant acknowledgements:
These studies were performed under NTNU Ph.D. grant project number 81771176, supported by Research Council of Norway project numbers: 250619 (Nano2021) and 272919 M-ERA-NET2/0007/2016-CellColor. Initial studies were performed under project NTNU Nanolab and the Norwegian Micro- and Nano-Fabrication Facility, project number 295864
The scholarship is to be given to a young researcher at a Nordic university, preferably at, or other similar strong collaborative link with Uppsala University to cover costs for a longer visit to a high ranking international research institute.
Application deadline: September 30, 2022

Maren Agdestein/NTNU
Jacob Lamb is an associate professor in sustainable energy systems in the department of energy and process engineering at NTNU. His research is focused on environmentally friendly energy storage technologies. Here he talks to John de Mello, Director of NTNU Nano, about life, research, and potential solutions to the climate crisis.
We are pleased to announce the launch of the NTNU Nano Expertise Directory – a simple search tool for finding out “who does what” in nanoscience at NTNU. The Directory covers around three quarters of our (permanent) nanoscience researchers, and we are actively working towards full coverage. In its current form, the Directory already provides the most detailed overview we have ever had of nanoscience at NTNU, and we hope you will find it a useful tool for navigating the wide range of nanoscience activities at NTNU. Try it out, and let us know what you think!
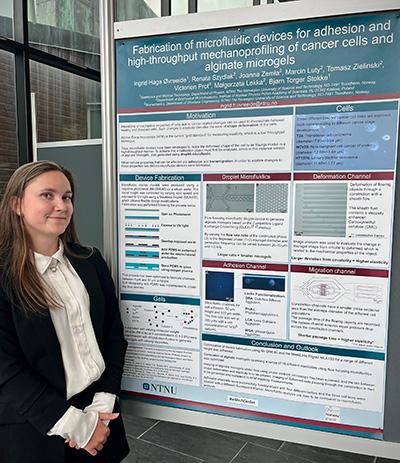
winning poster. Photo: Robert Karlson.
After two years of pandemic restrictions around 300 researchers in the Nordic Nanolabs Network could finally meet at Chalmers in Gothenburg on May 5-6, for the 3rd Nordic Nanolab User Meeting.
The meeting lasted for two days and included scientific tutorials, inspirational talks and networking. The programme also included a poster session with 75 contributions where NTNU NanoLab’s Ingrid Haga Øvreeide won one of the three poster prizes!
The meeting gathered participants from all five Nordic countries and twelve cleanrooms.
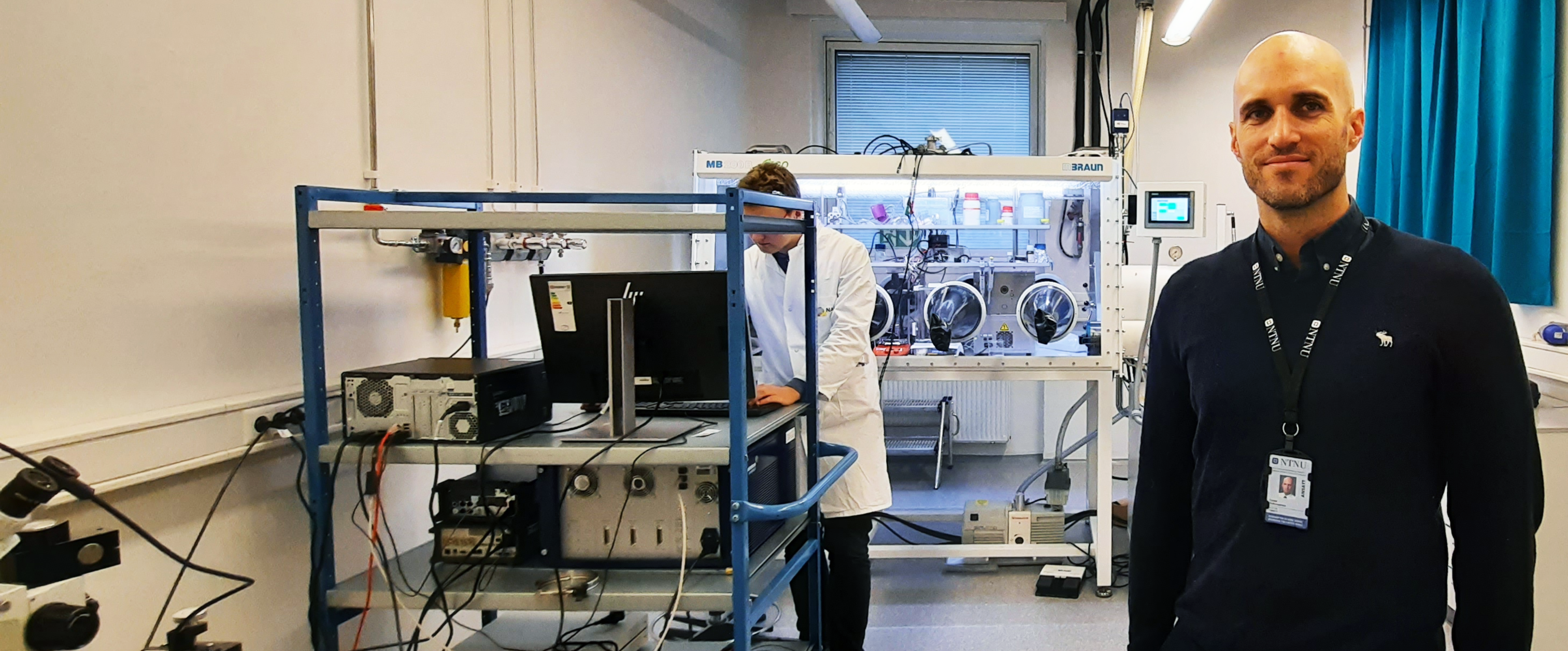
Batteries that can store more energy could help electric cars go the distance. But researchers at NTNU have discovered that one promising new battery material needs rethinking.
As drivers around the world switch to electric cars, new batteries that can store more energy, translating to longer driving distances before a car needs recharging, can’t come soon enough. But researchers at NTNU have discovered that one promising material in the search for the next generation of batteries needs rethinking.
Today, lithium ion batteries – the kind that are used in smartphones and other devices as well as electric cars – contain liquid electrolytes. Charged atoms known as ions move in one direction through the electrolyte, discharging an electron in order to power a device. To recharge the battery, ions move back through the electrolyte and regain an electron.
But batteries with liquid electrolytes are approaching their theoretical energy density limit. To store more energy without having to increase battery size, researchers are working on solid-state electrolytes instead. Solid-state batteries would be safer and more stable than their liquid electrolyte counterparts, making them useful in pacemakers and wearable devices, too.
Mixing ceramics with polymers
A polymer known as poly(ethylene oxide), or PEO, fits the bill as a solid electrolyte because it is flexible, light, and easy to process. But there’s a catch – PEO isn’t great at the one thing an electrolyte needs to do well: conduct lithium ions. Ceramic materials, on the other hand, conduct ions well but don’t have the mechanical advantages of polymers.
So, researchers began adding ceramic powders to the polymer as a “filler” material. “The idea was to put the ceramic particles inside these polymers, and somehow enjoy the best of both worlds,” says Daniel Rettenwander, an associate professor in the Department for Materials Science and Engineering at NTNU. In fact, many publications report that polymer electrolytes conduct ions better after adding fillers, even suggesting that the fillers form fast-track networks for lithium ions to move through the material.
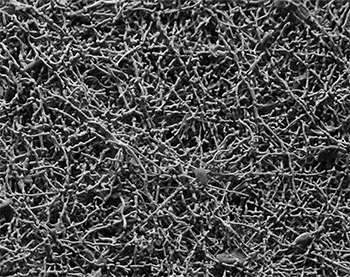
nanowires made of a lithium-oxide garnet
ceramic filler, credit: Rettenwander et al.
But in work published in the journal Frontiers in Energy Research, Rettenwander and colleagues have found that the fillers don’t actually play a part in transporting lithium ions in the electrolyte at all. While that may not sound like good news to those who were pinning their hopes on this simple combination of polymer and ceramic, the discovery could help nudge researchers down a more fruitful path that eventually leads to batteries we can use.
Understanding why it behaves the way it does
Rettenwander and colleagues made membranes with varying amounts of a lithium-oxide garnet ceramic filler known as LLZO, in the form of both particles and wires just nanometres in width. Then they looked at cross sections of the membranes using a scanning electron microscope and, in collaboration with Roland Brunner at the Materials Research Centre in Leoben, Austria, used x-ray computer tomography to take snapshots of the inside of the materials at the microscale.
Comparing those snapshots with measurements of the material’s properties meant the researchers could draw conclusions about how the filler particles affect the behaviour of ions in the polymer. ”We can understand why it behaves like it behaves,” says Rettenwander.
The researchers saw that both the particles and nanowires were evenly distributed throughout the polymer, and didn’t form networks that could fast-track lithium ions. The membranes with more of the filler were actually worse conductors of the ions, bolstering the conclusion that the filler doesn’t take part in transporting the ions.
“Just putting filler inside the membrane doesn't lead to any improvements,” says Rettenwander. “It's not the intrinsic property of the ceramic which improves the performance.”
So, why do some experiments find that adding fillers increases conductivity, if they’re not actually playing a part in transporting ions? Rettenwander thinks it could be the changes that occur in the polymer itself that gives the materials their advantage – going from an orderly crystal structure to a more amorphous, irregular pattern.
Could almost double energy density
This doesn’t mean that polymers with added filler are a dead end for research on solid state batteries. “The use of fillers is still a very good strategy, but it's just that it’s not enough to put the filler inside,” says Rettenwander. “You have to improve the interface between the polymer and the ceramic in order to make these membranes work.” He’s working on a way to help the materials bond better by changing their surfaces, for example.
If researchers can find a way to combine the best of both materials, solid state batteries for electric cars could significantly cut down on charging stops. “If you are able to make a solid state battery, then you will be able to almost double the energy density, increasing your driving distance almost by a factor of two,” says Rettenwander.
The project was funded by the Austrian Federal Ministry for Digital and Economic Affairs, the National Foundation for Research, Technology and Development and the Christian Doppler Research Association (Christian Doppler Laboratory for Solid-State Batteries)
Kelly Oakes, April 2022
Reference:
Role of Filler Content and Morphology in LLZO/PEO Membranes. Mir Mehraj Ud Din, M. Häusler, S. M. Fischer, K. Ratzenböck, Chamasemani, I. Hanghofer, V. Henninge, R. Brunner, C. Slugovc, and D. Rettenwander Front. Energy Res., 12 October 2021 https://doi.org/10.3389/fenrg.2021.711610
A list of NTNU papers related to nanoscience, nanotechnology and functional materials published in January - February 2022 may now found on NTNU Nano's page for publications.
Covering glass microscope slides in tiny, nano-sized pillars can mimic a cell’s natural environment – and could help biologists understand how cells act inside the human body.
When biologists study cells under a microscope, they look at them on flat surfaces that are nothing like the environment inside the human body. Now, researchers at NTNU have found a way to mimic some aspects of a cell’s native environment using tiny polymer pillars. Their work, funded by the Research Council of Norway, is published in the journal Nanoscale Research Letters.
While taking cells out of the extracellular matrix and putting them on flat surfaces made of glass allows researchers to study them in the lab, it does mean we could be missing out on observing many cellular processes.
“Glass is very hard and the cell will sense that the substrate does not deform as it tries to pull on it,” says Sikorski. “That induces certain types of behaviour and also induces certain types of processes in the cells. They will behave differently if they were placed on something which is elastic and soft and can be deformed and remodelled.”
This means that if researchers want to understand how cells behave in their native environment, they need a substrate which replicates biology more closely. Embedding cells within hydrogels – for example, 3D networks of gelatin-like polymers – is one option. But studying cells within a hydrogel isn’t as easy as looking at them on a simple glass slide under an optical microscope. “If you want to see what's happening it gets quite challenging,” says Sikorski.
Creating structures in a thin polymer film
Mimicking some of the mechanical aspects of softer substrates with nanostructures is one possible way to address this problem – and that’s exactly what Sikorski and PhD student Jakob Vinje have done, in collaboration with cell biologists Noemi Antonella Guadagno and Cinzia Progida at the University of Oslo.
Vinje covered glass slides in tiny pillars made of a polymer known as SU-8. These nanopillars – each measuring just 100 nanometres across at the tip – were made using electron beam lithography at NTNU NanoLab, where a focused beam of electrons creates structures in a thin polymer film.
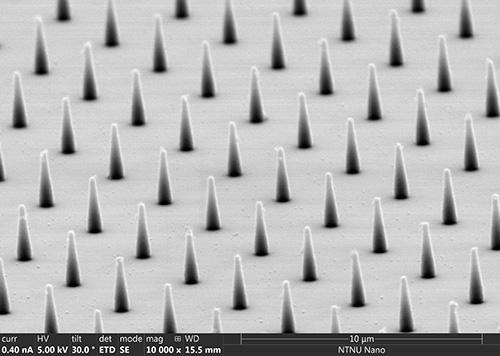
Photo: Jakob Vinje/NTNU NanoLab
“Per millimetre square you already have quite a lot of pillars, and if you want to study cells, then we need to make surfaces which are at least on the order of 10 by 10 millimetres,” says Sikorski. “The tools in NTNU NanoLab are essential for this to be possible.”
The researchers created substrates with a variety of different nanopillar arrangements and tested them using cells which produce fluorescent proteins. Looking at the cells under a microscope, the researchers analysed the shape, size and distribution of the points at which the cell attaches to the different surfaces.
Tightly packed pillars
After making hundreds of observations of cells on the various surfaces, the researchers found that substrates with tightly packed nanopillars most closely mimicked a softer surface. “If we make a substrate with dense pillars then the cells behave as if they were on a much softer substrate,” says Sikorski.
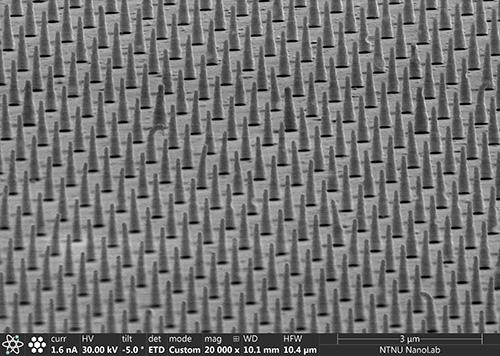
Photo: Jakob Vinje /NTNU NanoLab
The beauty of the nanopillar-covered substrates is their simplicity – in theory, biologists could simply swap their usual glass slides for the new ones. “It has more features and more tunability than a glass substrate, but it's still relatively simple,” says Sikorski.
He says the ultimate aim would be for researchers to be able to ”just open the package and take one of them out, put their cells on, study it under the microscope and then throw it away once they're done.” However, for that to become a reality the substrates would need to be produced in their hundreds at a relatively low cost.
So far the researchers have only made a small number of prototypes, but there are existing methods – such as a low-cost, high-throughput technique for making nanoscale patterns called nanoimprint lithography – that could make scaling up production of the substrates possible.
As well as allowing biologists to study cells in a new way, the substrates could be used to develop better ways to screen medicines. To find a drug that stops cells sticking to a particular surface, for example, a nanopillar-covered substrate could mimic that surface and put potential medicines to the test.
Kelly Oakes, February 2022
The research was funded by Research Council of Norway Grant No. 295864
Reference:
Vinje, J.B., Guadagno, N.A., Progida, C. et al. Analysis of Actin and Focal Adhesion Organisation in U2OS Cells on Polymer Nanostructures. Nanoscale Res Lett 16, 143 (2021). https://doi.org/10.1186/s11671-021-03598-9
News 2021
This year, the Norwegian NanoSymposium will be organized in conjunction with the first two days of the kick-off conference for the Research School for Training the Next Generation of Micro- and Nanotechnology Researchers in Norway (TNNN).
The whole event takes place at Akrinn in Trondheim on November 30th – December 2nd, 2022.
The program will include plenary and invited talks from leaders in various areas of nanotechnology, contributed talks from PhD candidates and post doctoral researchers, presentations from industry, workshops and networking events.
No conference fee will be charged from academic participants. However, registration is mandatory. The deadline for registering and submission of abstracts for poster and oral presentations is November 1st.
More information about the conference program and registration can be found on the TNNN Conference home page.
Have you not registered as a member of TNNN yet?
Information about the TNNN research school and registration for membership may be found on the TNNN home page.
Don’t miss this excellent opportunity to make new contacts with the Norwegian nanoscience community.
The Research School for Training the Next Generation of Micro- and Nanotechnology Researchers in Norway (TNNN) will address current gaps in PhD-level education in this field. In particular, it will establish a vibrant national network of junior scientists working in this area of science and technology development, provide training in transferable skills and facilitate collaboration with industry. TNNN will contribute both to raising the quality of Norwegian micro and nano research and to making PhD education in MNST more relevant.
Read more about TNNN and register as a member on the TNNN homepage.
The Rector of NTNU has allocated four PhD-positions to each of the following areas:
• Biotechnology
• Nanotechnology, nanoscience and functional materials
• Digital technologies (information and communication technology)
The positions will be allocated to highly innovative projects that have clear potential to contribute to NTNU’s ambition of substantially increasing the number of major national and international research grants. We would like to particularly encourage early career researchers who have ambitions applying for ERC starter/consolidator grants to apply for the PhD-positions.
Deadline for proposals: 30th of January 2022
Scientific staff at NTNU are invited to submit research proposals by completing this online application form.
Note: It is not possible to save the form for later editing. We therefore recommend that you use our proposal template while you develop the proposal.
Specific requirements for proposals involving:
Nanotechnology, nanoscience and functional materials
At least two of the positions will be allocated to experimental projects involving work at NTNU NanoLab. The PhD students associated with these two positions will be expected to carry out duty work in the general area of process development and competence building within nano-structuring, in particular thin-film processing and lithography. They may also be required to contribute to training of Master students within the lab. There are no restrictions on the other two projects, which may be of a theoretical or experimental nature, but the students will be expected to contribute to duty work in a related area in the NanoLab where possible. We particularly encourage applications that involve open science, advanced nanofabrication techniques, or interdisciplinary research at the health/engineering or health/natural-sciences interface.
Biotechnology
Only interdisciplinary projects that address topics in accordance with the definition of biotechnology used by Norwegian authorities will be considered, i.e. the application of science and technology to living organisms as well as parts, products and models thereof, to alter living and non-living materials for the production of knowledge, goods and services.
Digital technologies
Proposals addressing fundamental issues in information and communication technology (ICT) and in the application of digital technology are particularly welcome. The proposals aligned with NTNU Digital’s strategic research such as Artificial Intelligence, Cyber Security and Reliability, IoT and others will be particularly welcomed. Projects containing cross-disciplinary research cooperation will be favourably regarded, as will support from other sources, e.g. matching funding/positions from the applicants' faculty.
Evaluation criteria
Information about the evaluation criteria for the proposals.
More information
Questions regarding the announcement may be sent to John de Mello and Hanna Gautun
Countless potentially useful enzymes are hidden all around us. Now a team of researchers at NTNU have developed a new method that could help us find them.
The natural world is a treasure trove of potentially useful enzymes, hidden in microorganisms living all around us. But finding them is tricky. A team of researchers at NTNU have developed a new method to break open cells that could help in the quest.
Enzymes are biological catalysts that can make industrial processes cheaper, less toxic, and more sustainable – and researchers are discovering new ones all the time. For example, a bacteria that uses two enzymes to break down plastic was found outside a plastic bottle recycling facility by Japanese researchers in 2016. “Nature has a vast capacity for producing enzymes,” says Rahmi Lale, a synthetic biologist at NTNU’s Department of Biotechnology and Food Science.
Now, Lale and his colleagues have developed a new technique to break open cells in a controlled way – a vital step in screening for helpful enzymes. Crucially, the method leaves some cells intact, allowing them to be recovered and investigated further. The work, partly funded by the Research Council of Norway and the EU HORIZON 2020 programme, has been published in the journal ACS Synthetic Biology.
Millions of different cells
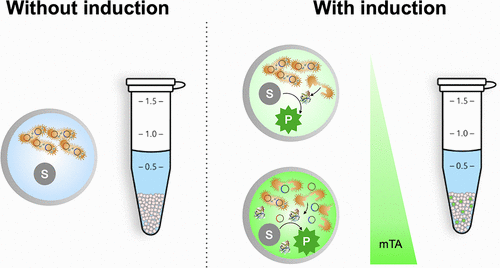
(Credit: Wong et al. 2021) https://pubs.acs.org/doi/10.1021/acssynbio.1c00084
To find a new enzyme in nature, the first step is to identify an environment where microbes that need such an enzyme might live. Heat-resistance is likely to be prized in the desert, for instance, whereas carbohydrate-degrading enzymes are more likely to be found in the human gut. Next researchers sift through material from that environment, such as a soil sample, to find genetic material. After isolating this environmental DNA, researchers cut it up into smaller pieces in the lab and paste it into well-studied microorganisms like E. coli (a process known as cloning) to screen
for enzymatic reactions
This leads to countless microbes, each of which could contain what the researchers are looking for. From this point the search becomes a “needle in a haystack problem,” says Lale. “It's easy to collect DNA, it's easy to clone them, and it's easy to introduce them into these microorganisms,” he says. “But all of a sudden you have hundreds of millions of different cells. Every one of them carries something unique, but you don't know which carry things that you are interested in.”
Screening the candiates
Using microfluidics – liquids flowing through tiny channels etched into a chip – allows researchers to screen the microbes 10,000 times faster than previous methods. The microbes are contained in water droplets that sit within an oil-based carrier fluid. But because most of the potentially useful enzymes are made inside those microbes, the cells have to be opened up in order to test them.
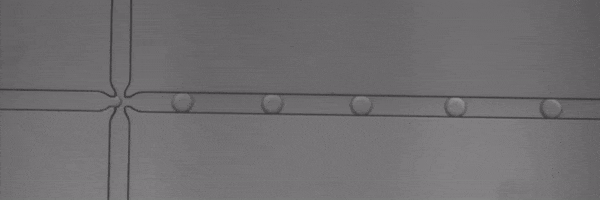
To do this, Lale and his colleagues have developed a system that deliberately punctures the membrane of a cell, so its insides leak out into its surroundings. There, it can be tested for the presence of whatever kind of enzyme the team are looking for. “We have a substrate that waits for the enzyme to come and interact with it,” says Lale. If there’s a positive match, it will trigger a reaction.
Controlling the holes
The standard way to break down the membrane of a cell, a process known as lysis, involves a chemical solution that is all but guaranteed to kill every cell in the droplet. But this presents a problem for researchers who, after finding an enzyme of interest, want to probe the microbe it came from further.
In contrast to chemical lysis, the “lysis-on-demand” system can be controlled by adjusting the concentration of the substance that induces lysis, meaning the researchers can deliberately leave some cells intact so they can be recovered later on. “Because we can control how many holes we’re introducing, we can also control how many cells die,” says Lale. “We’re not killing them all, and that’s important.”
“If something of interest happens in one particular droplet, then we can recover that droplet,” he says. “Thanks to cells growing so quickly, we can take the droplet, put it in a growth medium and the next day have a billion cells again. Then recovery of DNA becomes a really simple task.” The researchers confirmed their new technique in microfluidic chips they made in NTNU NanoLab.
From northern Norway to a UK compost heap
As well as boosting the search for useful molecules in nature, the technique could give a helping hand to researchers attempting to steer microbes towards making enzymes with particular traits. By introducing genetic mutations in the lab, researchers can nudge the microbe in numerous different directions – then use microfluidic screening combined with the lysis-on-demand system to find the microbe that was nudged closer to making the kind of enzyme they want.
Lale and his colleagues have applied for a grant to explore this aspect of the research further, as part of a consortium led by colleagues at the University of Cambridge, UK. The researchers plan to use the system to sift through environmental DNA samples from various locations that could yield interesting enzymes, including the cold of northern Norway, the hot climate of southern Spain, and a UK compost heap. “If you look into these interesting environments, the chances of finding something is higher,” says Lale.
Kelly Oakes, November 2021
The research was funded by:
he Research Council of Norway Grant No. 295864 and EU HORIZON 2020 programme Grant No. 685474
Reference:
Che Fai Alex Wong, Liisa van Vliet, Swapnil Vilas Bhujbal, Chengzhi Guo, Marit Sletmoen, Bjørn Torger Stokke, Florian Hollfelder, and Rahmi Lale. A Titratable Cell Lysis-on-Demand System for Droplet-Compartmentalized Ultrahigh-Throughput Screening in Functional Metagenomics and Directed Evolution. ACS Synth. Biol. 2021, 10, 8, 1882–1894. July 14, 2021. https://doi.org/10.1021/acssynbio.1c00084
A list of NTNU papers related to nanoscience, nanotechnology and functional materials published in October 2021 may now found on NTNU Nano's page for publications.
Ine Larsen Jernelv defended her thesis “Mid-Infrared Tuneable Laser Spectroscopy for Glucose Sensing” in August 2020. The doctoral work was carried out at the Department of Electronic Systems, where Professor Astrid Aksnes was her supervisor and Professor Dag Roar Hjelme was her co-supervisor.
The Dimitris N. Chorafas Foundation awards scientific prizes for outstanding work in selected fields in the engineering sciences, medicine, and the natural sciences. It rewards research characterized by its high potential for practical application. NTNU has been a member institution since 2013.
The committee for the Chorafas Prize praised the thesis:
The thesis appears complete and compact representing a body of independent work
conforming to the highest academic standards. Without doubt this thesis is equivalent to an
excellent thesis at any international university, and would not only have been approved, but
would have received the highest grade.
To describe the research project, here are the candidate’s own words:
"The focus of my research was on biomedical applications of mid-infrared spectroscopy, specifically for glucose sensing. Mid-infrared sensing is a versatile analytical technique, with recent technological developments that enable further advancements towards miniaturised and portable sensor applications. The aim of my thesis work was to develop an experimental setup and analytical methods for fast and accurate measurements of glucose in biological fluids.
Glucose sensing is a critical tool for management of diabetes, and current commercially available devices that measure subcutaneously have shortcomings such as lag time compared to the actual blood glucose level. Fluid measurements in the peritoneal cavity in the abdomen have been suggested as a possible replacement for subcutaneous monitoring. While previous research has investigated mid-infrared spectroscopy measurements for hospital settings or non-invasive monitoring, there has been less interest in solutions targeted towards portable sensing. This work was therefore concentrated on a fibre-coupled sensor system with a quantum cascade laser (QCL) source, with development towards a continuous monitoring (CGN) device."
Ine’s research has resulted in the software package SpecAnalysis to analyse spectroscopy data, made available for free on GitHub. More importantly, her research may now be used to make better glucose measurement implant for humans. The lag times can now be only 10 seconds, as opposed to the 10 – 15 minutes that we currently have. Better implants have the potential to improve health care and daily life for diabetics.
Ine is currently working as a researcher at the Arctic University of Norway in Tromsø. On 3 December, she will come to NTNU in Trondheim to receive the award and will also hold a lecture in EL5. Title to be announced.
We send her our heartfelt congratulations for the well-deserved Chorafas prize!
A list of NTNU papers related to nanoscience, nanotechnology and functional materials published in September 2021 may now found on NTNU Nano's page for publications.
Researchers at NTNU have developed a new elastomer with unprecedented stiffness and toughness, inspired by spider silk
Inspired by extremely strong spider silk, researchers at NTNU have developed a new material that defies previously seen trade-offs between toughness and stiffness.
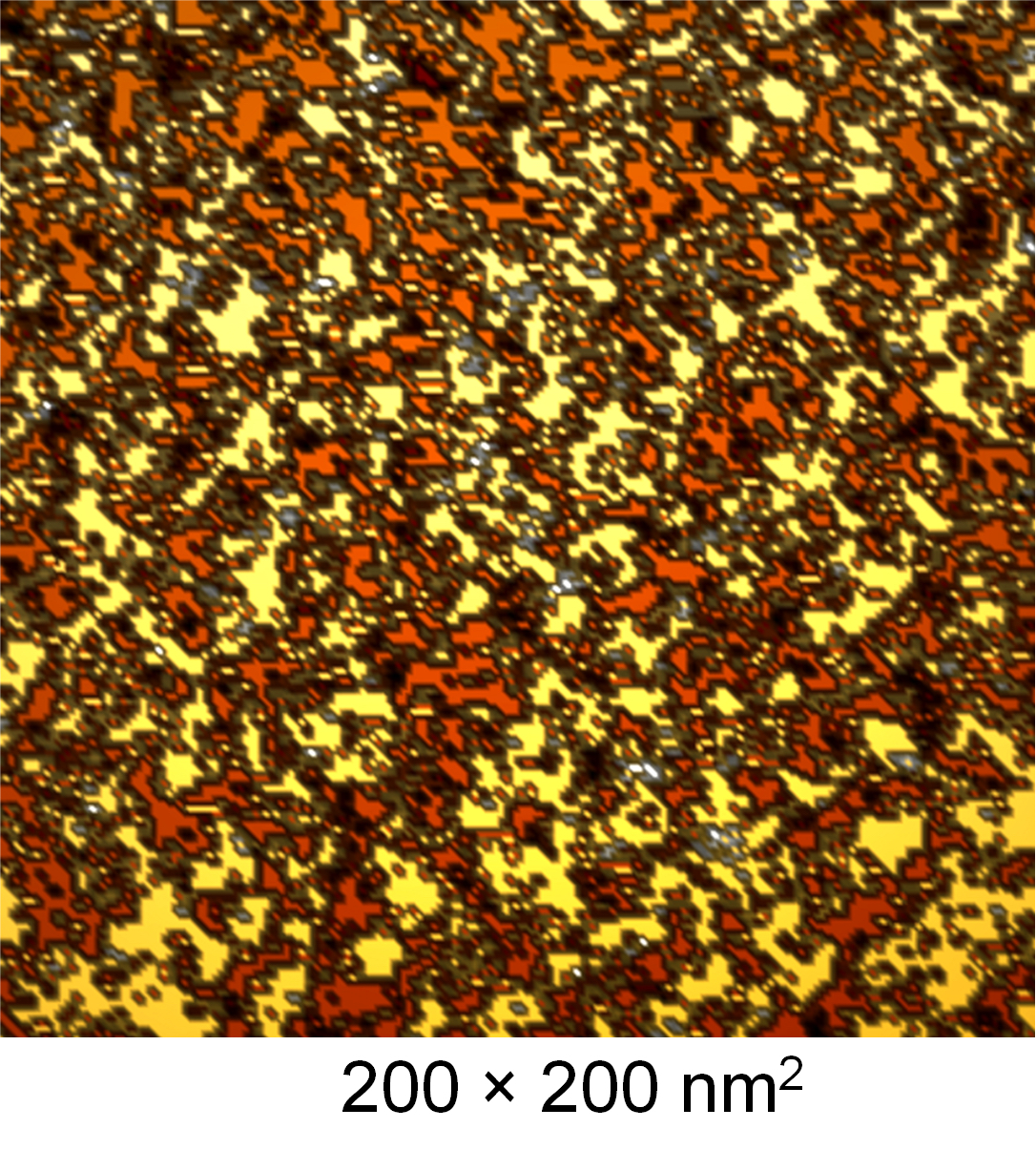
and soft domains using an atomic
force microscope.
Credit: Zhang et al (2021)
The material is a type of polymer known as an elastomer because it has a rubber-like elasticity. The newly developed elastomer features molecules that have eight hydrogen bonds in one repeat unit, and it is these bonds that help to evenly distribute stress put on the material and make it so durable.
“The eight hydrogen bonds are the origin of the extraordinary mechanical properties,” says Zhiliang Zhang, professor of mechanics and materials at NTNU’s department of structural engineering. The material was developed at NTNU NanoLab and partially funded by the Research Council of Norway.
The idea to introduce a higher than usual number of hydrogen bonds came from nature. “Spider silk contains the same kind of structure,” says Yizhi Zhuo, who developed the new material as part of his PhD and postdoc work. “We knew it could result in very special properties.”
Scientists have previously noted that spider silk – specifically dragline silk, which provides the spokes and outer rim of a spider’s web – is both exceptionally stiff and tough.
Stiffness and toughness are distinct properties in engineering and are often in opposition. Stiff materials can withstand a lot of stress before deforming, whereas tough materials can absorb a lot of energy before they break. Glass, for example, is stiff but not tough.
Until now, replicating the dual stiffness and toughness of spider silk in synthetic elastomers has not been possible. “With commercial materials, if you want to have higher stiffness, you have lower toughness. It’s a trade off. You cannot have both,” says Zhang.
The team’s new elastomer features distinct hard and soft domains. After devising and making it, the team used an atomic force microscope – with a resolution of fractions of a nanometre – to look at the underlying structure of the material, and observe the interface between the hard and soft regions.
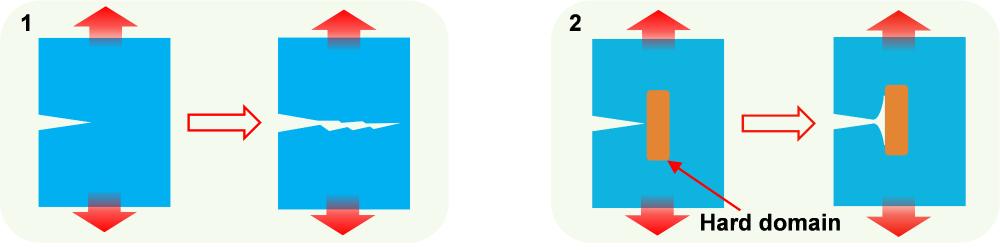
They saw that as well as the eight hydrogen bonds distributing stress, the mismatch in stiffness between the hard and soft domains helped to dissipate energy further by encouraging any cracks to branch off instead of continuing along a straight path. “If you have a zig-zag, you create a large fracture surface and dissipate more energy, so you have higher toughness,” says Zhang.
Alongside it’s mechanical properties, the material is optically transparent and research suggests it could even self-heal at temperatures higher than 80 °C. If production can be scaled up, the new material could one day be used in flexible electronics – particularly wearable devices that are more prone to damage and breakages.
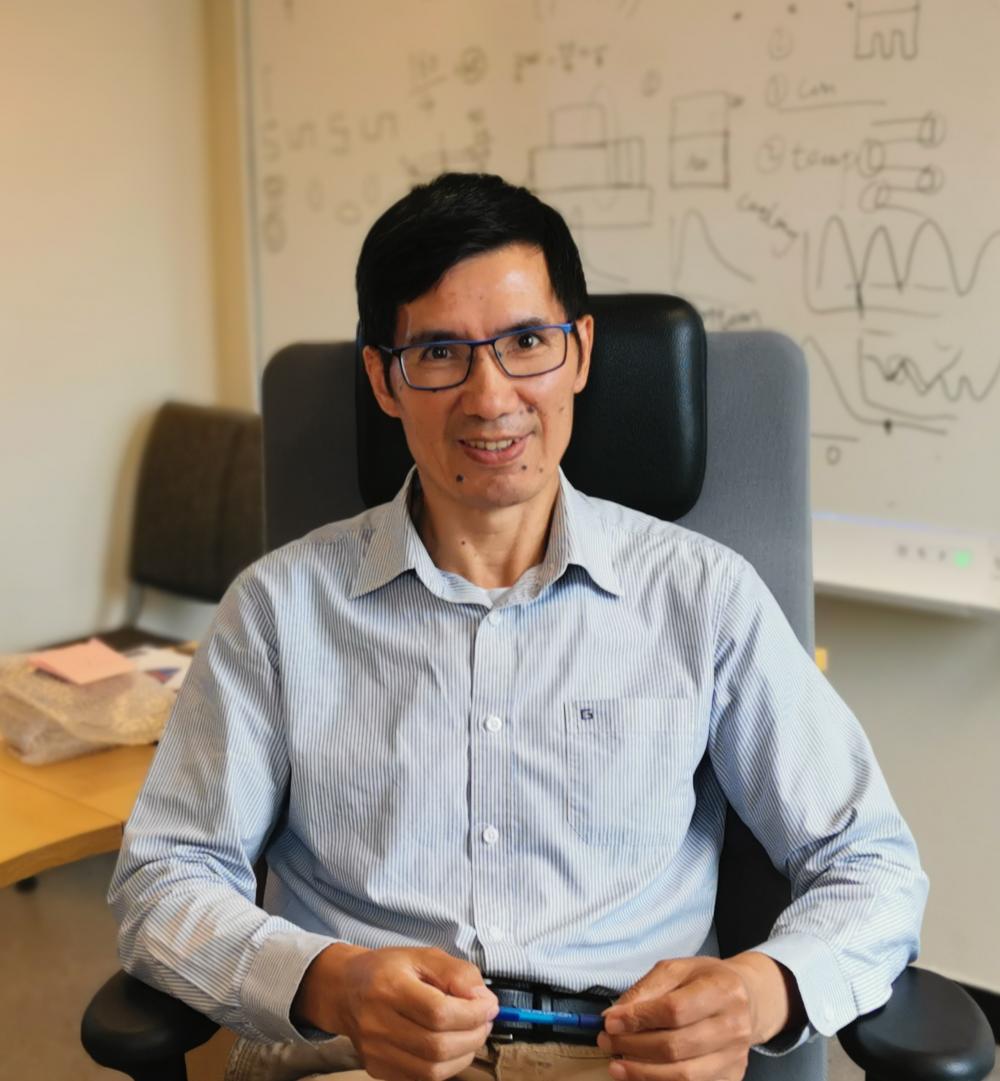
Photo: Jianying He
Zhang and his colleagues filed a patent for their material in March, but they continue to work on introducing other desirable properties to it. The soft domains in their material are made up of a silicon-based polymer known as PDMS, but the researchers suspect they could improve the mechanical properties even further by experimenting with other substances.
They would also like to extend the material’s properties to include anti-icing – stopping ice sticking to it at low temperatures – and anti-fouling – preventing aquatic organisms like mussels and algae attaching to it – so it could be used in extreme conditions, such as the Arctic. “This material is a good starting point, but we want to add some other functionality,” says Zhang.
Kelly Oakes, September 2021
The research was funded by Research Council of Norway grant numbers 255507 and 245963.
Reference: Zhuo, Y., Xia, Z., Qi, Y., Sumigawa, T., Wu, J., Šesták, P., Lu, Y., Håkonsen, V., Li, T., Wang, F., Chen, W., Xiao, S., Long, R., Kitamura, T., Li, L., He, J., Zhang, Z., Simultaneously Toughening and Stiffening Elastomers with Octuple Hydrogen Bonding. Adv. Mater. 2021, 33, 2008523. https://doi.org/10.1002/adma.202008523
A list of NTNU papers related to nanoscience, nanotechnology and functional materials published in June - August 2021 may now found on NTNU Nano's page for publications.
No conferences to travel to this year? Tired of watching long, long lectures on Zoom?
Then this year’s Norwegian NanoSymposium might be just the thing for you! The NanoSymposium will be a very different kind of on-line meeting, with a dynamic mix of invited speakers, short Pecha Kucha presentations, and plenty of breaks and interactive sessions. This is an excellent opportunity to learn a little about the parts of nanoscience that you don't get to study or research every day.
Our aim is to hold a fun meeting covering all areas of nanoscience, where students and researchers from many disciplines can share ideas and make new contacts.
What is a Pecha Kucha?? Glad you asked...
Pecha Kucha is a concentrated pitch where the participants present exactly 20 slides at a rate of 20 seconds per slide. The entire presentation lasts 6 minutes and 40 seconds. You may find information about the Pecha Kucha format and how to prepare one here and here. Be sure to check out the examples for inspiration!
Thanks to sponsorship from NTNU Nano, there is no participation fee, but you will need to register your attendance.
Researchers at NTNU are studying brain cells in the lab to investigate the foggy beginnings of diseases like Parkinson’s and Alzheimer’s.
When the symptoms of neurodegenerative diseases like Parkinson’s become clear enough to make a diagnosis, there have already been significant changes in a person’s brain. That’s why researchers believe that finding a way to identify this turning point could be the key to better treatments.
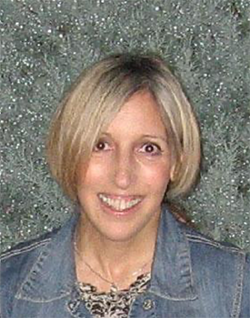
Angella Niarou
“In theory, if you can pinpoint the onset of the disease, you might be able to stop it or reverse some of its effects,” says Ioanna Sandvig, co-leader of the integrative neuroscience group at NTNU’s Department of Neuromedicine and Movement Science. “By the time you actually have very strong indications that something is wrong, then it's a bit too late.”
Sandvig and her colleagues are growing interconnected brain cells in the lab, to study how these neural networks evolve and what happens when things go wrong. The research, supported by the NTNU program for Enabling Technologies, could help to pinpoint the very beginnings of neurodegenerative diseases.
Chip that mimics connectivity
Each neural network contains bundles of neurons – the cells that carry messages in our brains – housed in a microfluidic chip, the prototype of which was designed by Rosanne van de Wijdeven during her PhD at NTNU. These nodes are connected by tunnels in the chip through which axons – the wire-like protrusions of a neuron – can grow, but the main body of a neuron cannot.
By connecting three nodes together the researchers can mimic the connectivity inside the human brain. But Sandvig is keen to stress that these neural networks are not in any way real brains. “We don’t have brains in the lab,” she says. “But we do have networks that are representative, and are very malleable to the perturbations we want to introduce.”
The chips contain microelectrode-arrays made in NTNU’s NanoLab that enable the researchers to measure the electrical activity of the network and gain insight into how signals are passing between the neurons.
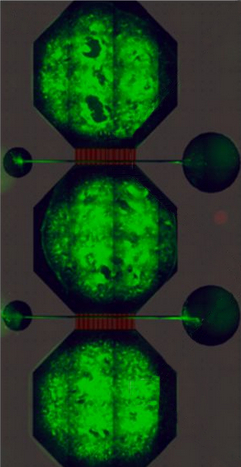
fluidic chip. Photo: NTNU
In one recent study, led by Vibeke Devold Valderhaug and detailed in a paper uploaded to the pre-print server bioRxiv, the researchers cultivated two groups of neural networks in parallel, one of which housed neurons with a gene mutation that has been linked to Parkinson’s disease known as LRRK2 G2019S. They saw that, in the network with the mutation, neurons grew and formed connections in a markedly different way, and displayed different electrical activity, to the healthy network.
Neurons typically navigate their environment in a very specific manner, says Sandvig, but in the network with the mutation the cells didn’t have the kind of directionality you would expect – they seemed confused. “The LRRK2 mutated networks seem to have some kind of aberrant growth,” says Sandvig. “They seem to interpret exactly the same cues – because it's the same substrate – in a totally different way than the healthy network.”
As well as those findings, Sandvig was pleased to see how clearly the subtle changes showed up in the team’s experimental set up. “What was surprising was how well we could pick it up with this interface,” she says. The interface also allowed the researchers to look at the connectivity within the nodes as well as between them.
Studying these brain changes in a neural network has advantages over studying them in animals, though each method can inform the other. “You can have these snapshots of changes in the structure and function of the network much more easily than in animal models,” says Sandvig.
With neural networks, it’s also possible to take cells from the same individual and derive them in two separate ways to compare how the networks evolve when age-related effects are removed. In fact, one of the follow up projects Sandvig is developing with integrative neuroscience group co-leader Axel Sandvig alongside colleagues at the Kavli Institute for Systems Neuroscience does exactly this for Alzheimer’s disease. “Diseases like Parkinson's, ALS, Alzheimer's are all different, but they share some very fundamental characteristics,” she says.
By providing new insights into how our brains change in the early days of neurodegenerative disease, real life neural networks could set us on a path to new understanding, and perhaps, eventually, even new treatments.
Kelly Oakes, June 2021
RCN project number: 295864 (NorFab)
A list of NTNU papers related to nanoscience, nanotechnology and functional materials published in May 2021 may now found on NTNU Nano's page for publications.
Researchers from NTNU are shedding light on magnetic materials at small scales by creating movies with the help of some extremely bright x-rays.
Erik Folven, co-director of the oxide electronics group at NTNU’s Department of Electronic Systems, and colleagues from NTNU and Ghent University in Belgium set out to see how thin-film micromagnets change when disturbed by an outside magnetic field. The work, partially funded by NTNU Nano and the Research Council of Norway, was published in the journal Physical Review Research.
Tiny magnets
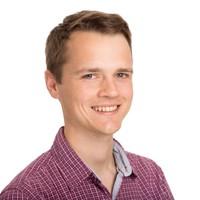
photo: Kai T. Dragland.
The tiny square magnets, created by NTNU PhD candidate Einar Digernes, are just two micrometers wide and split into four triangular domains, each with a different magnetic orientation pointing clockwise or anti-clockwise around the magnet. These domains meet at a central point – the vortex core – where the magnetic moment points directly in or out of the plane of the material.
“When we apply a magnetic field, more and more of these domains will point in the same direction,” says Folven. “They can grow and they can shrink, and then they can [merge] into one another.”
Electrons almost at the speed of light
Seeing this happen isn’t easy. The researchers took their micromagnets to an 80m-wide donut-shaped synchrotron, known as BESSY II, in Berlin, where electrons are accelerated until they are travelling at almost the speed of light. Those fast moving electrons then emit extremely bright x-rays. “We take these x-rays and use them as the light in our microscope,” says Folven.
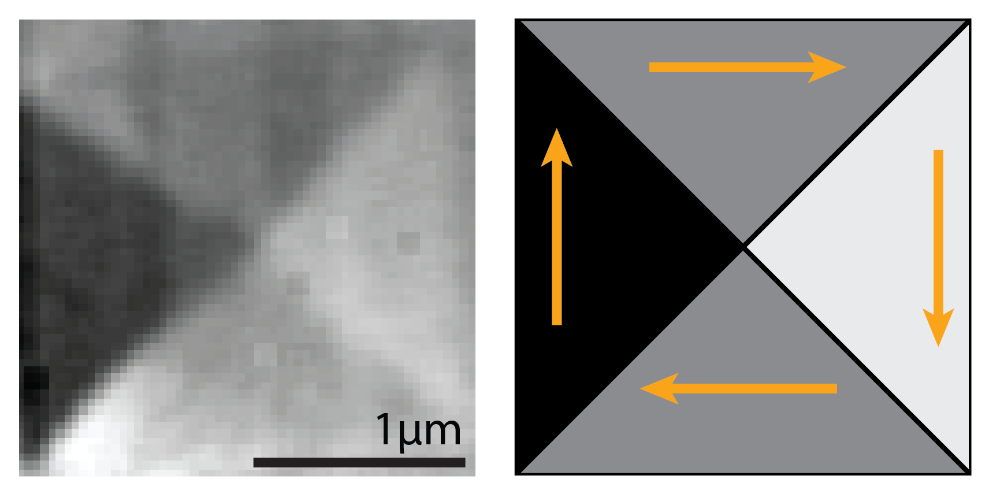
Photo: Einar Digernes, NTNU
Because electrons travel around the synchrotron in bunches separated by two nanoseconds, the x-rays they emit come in precise pulses. A scanning transmission x-ray microscope, or STXM, takes those x-rays to create a snapshot of the material’s magnetic structure. By stitching these snapshots together, the researchers can essentially create a movie showing how the micromagnet changes over time.
With the help of the STXM, Folven and his colleagues disturbed their micromagnets with a pulse of current that generated a magnetic field, and saw the domains change shape and the vortex core move from the centre. “You have a very small magnet, and then you poke it and try to image it as it settles again,” he says. Afterwards, they saw the core return to the middle – but along a winding path, not a straight line. “It will kind of dance back to the centre,” says Folven.
One slip and it’s over
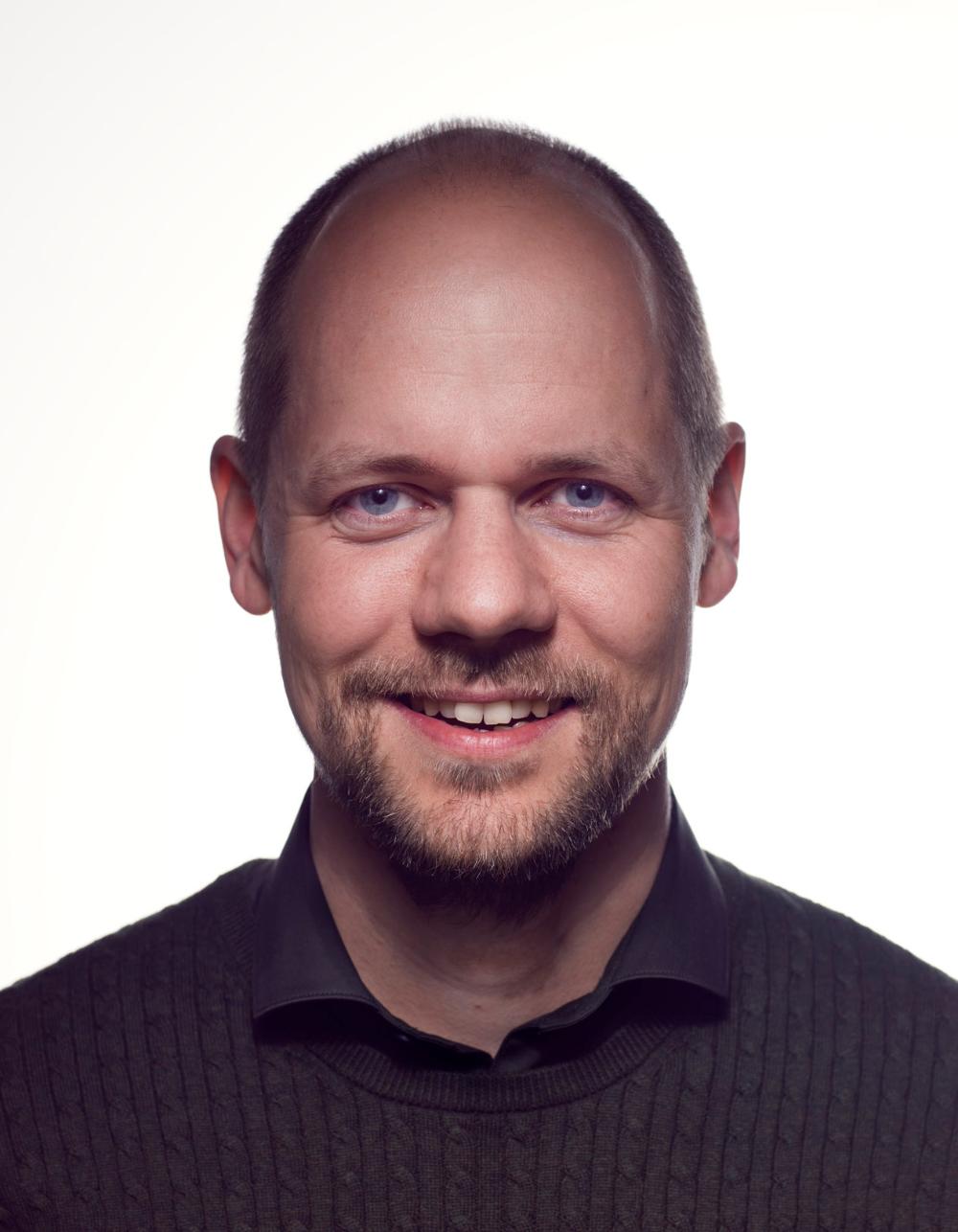
Terje Trobe
The team’s biggest challenge was making the samples in the first place. “We were very, very uncertain whether it would even be possible when we started out,” says Folven.
That’s because they study epitaxial materials, which are created on top of a substrate that allows researchers to tweak the properties of the material, but would block the x-rays in a STXM.
To solve the substrate problem, the researchers buried their micromagnet under a layer of carbon to protect its magnetic properties. Then they carefully and precisely chipped away the substrate underneath with a focused beam of gallium ions until only a very thin layer remained. The painstaking process could take eight hours per sample – and one slip up could spell disaster.
“The critical thing is that, if you kill the magnetism, we won't know that before we sit in Berlin,” he says. “The trick is, of course, to bring more than one sample.”
From fundamental physics to future devices
Thankfully it worked, and the team used their carefully-prepared samples to chart how the micromagnet’s domains grow and shrink over time. They also created computer simulations to better understand what forces were at work.
As well as advancing our knowledge of fundamental physics, understanding how magnetism works at these length and time scales could be helpful in creating future devices.
Magnetism is already used for data storage, but researchers are currently looking for ways to exploit it further. The magnetic orientations of the vortex core and domains of a micromagnet, for example, could perhaps be used to encode information in the form of 0s and 1s.
The researchers are now aiming to repeat this work with anti-ferromagnetic materials, where the net effect of the individual magnetic moments cancels out. These are promising when it comes to computing – in theory, anti-ferromagnetic materials could be used to make devices that require little energy and remain stable even when power is lost – but a lot trickier to investigate because the signals they produce will be much weaker.
Despite that challenge, Folven is optimistic. “We have covered the first ground by showing we can make samples and look through them with x-rays,” he says. “The next step will be to see whether we can make samples of sufficiently high quality to get enough signal from an anti-ferromagnetic material.”
Kelly Oakes, May 2021
RCN project numbers: 221860 and 295864
A list of NTNU papers related to nanoscience, nanotechnology and functional materials published in April 2021 may now found on NTNU Nano's page for publications.
As sunlight filters through a forest canopy, chlorophyll is hard at work capturing the energy of photons. Inspired by nature, researchers at NTNU are working on light-capturing dyes for solar cells to generate electricity.
These aren’t the kind of solar cells you’ll see on the roof of a building. In those silicon solar cells, light hits one of two semiconductor layers and frees up electrons to jump between the layers. It’s the movement of these electrons that creates an electrical current. A dye-sensitised solar cell (DSSC) works in a similar way, but one of the semiconductor layers is replaced with a photosensitive dye that absorbs the light and releases electrons instead.
Dye-sensitised solar cells tend not to be as efficient at converting light into electricity as their silicon counterparts. But they work in low light conditions, and can be transparent and flexible, so are better suited to some applications. To really take full advantage of DSSCs, a research project partially funded by the Research Council of Norway (RCN)* is looking for ways to step up their efficiency.
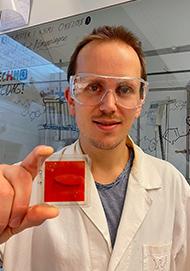
photo: Vilde Bråten
In a paper published recently in the journal Dyes and Pigments, NTNU PhD candidate David Moe Almenningen and colleagues, Odd Reidar Gautun, Bård Helge Hoff and Svein Sunde have shown that adding a particular molecule to the dyes can increase its light harvesting properties – though so far the additional light comes at a cost.
To harvest light a dye needs to act as an electron donor and an electron acceptor. “When this molecule is struck by a ray of sunlight, then the electron moves from the electron-rich part to the electron-poor part,” says Almenningen. By adding something in-between the donor and acceptor, chemists are able to increase the amount of light the cell harvests.
Almenningen’s research is investigating the addition of compounds featuring thiophenes, a molecule similar to benzene but containing sulphur. Thiophenes are electron-rich, so would be expected to increase the light harvesting properties of the dye, he says. And recent experiments show that they do: the dye with the most thiophenes was the one that harvested most light.
However, it turns out that increasing the amount of light a dye captures doesn’t automatically mean better solar cells. Put simply: you might get more electrons, but they don’t necessarily go where you want them to.
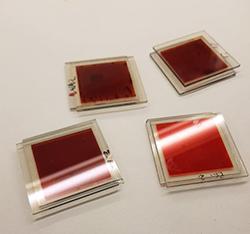
photo: David Moe
Almenningen
In his experiments, Almenningen found that though it absorbed the most light, the dye with the most thiophenes actually made the least efficient solar cell. “You think you're doing something brilliant by increasing the light harvesting ability, but then there are other reactions going on in the solar cell that are negatively affected by these modifications,” he says.
He and his colleagues hope to find a way to avoid those counterproductive effects and take advantage of the improved light collection. Their next step is to try modifying the dye chemically so the electrons can only go in one direction. If this is successful, it could lead to more efficient solar cells.
Finding a way to increase the efficiency of DSSCs is one of the roadblocks to widespread use. The current highest efficiency is around 12%, compared with closer to 20% for a traditional commercial silicon solar cell.
If researchers are able to harness the light captured by dyes in these solar cells more effectively, DSSCs would potentially offer an advantage over traditional crystalline solar cells when it comes to scaling up: they are cheap to make, because they don’t need a clean room or vacuum technology.
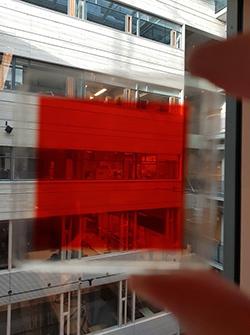
be used as part of a building’s
window or facade. Photo:
David Moe Almenningen.
One promising avenue for DSSCs would be to integrate them into buildings to capture the dimmer light that is typically found indoors. “That’s where these solar cells shine,” says Almenningen. “Also they look quite pretty. You can customise any colour you want, they can be see-through.”
For Almenningen, though, the reward is in figuring out how changing the chemical structure affects the performance of the dye: “The chemistry in itself is what's fascinating.”
*RCN project numbers: 262152, 226244 and 295864
Kelly Oakes, March 2021
A list of NTNU papers related to nanoscience, nanotechnology and functional materials published in March 2021 may now found on NTNU Nano's page for publications.
Modern-day computers rely on the fact that electrons have charge. But electrons have another fundamental property called spin – a measure of magnetic orientation – that researchers hope to harness to create a new generation of computer chips. Spintronics – short for spin transport electronics – could lead to faster, more stable, and less power-hungry devices.
An electron’s spin is a bit like a compass needle that points north or south. Magnetic hard drives already use the spin of electrons to store information in the form of binary 0s and 1s, which your computer can then translate back into human-readable information. But traditional computer processing ignores spin entirely. Using spin for computation would mean processing and storage could happen on the same chip.
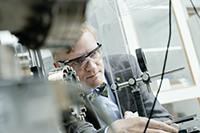
Photo: Geir Mogen
In most materials, there are equal numbers of electrons with spins that point in opposite directions, so from the outside they all appear to cancel out. These materials are known as antiferromagnetic, and Thomas Tybell, a professor in the department of electronic systems at NTNU and his colleagues are looking for ways to engineer them for use in future spintronic devices. “If we use antiferromagnetic materials, where the spins cancel, they are very robust against perturbations,” he says.
That stability is a big plus. Let’s say you’re working on an important document, and just before you hit save there’s a power cut. With conventional computing, you have probably just lost your work. But the spin of an electron stays the same even when the power is lost, so on a spintronic computer your work would be preserved.
But to create spintronic devices, we first need materials that allow us to reliably control spin.
One big challenge is engineering materials without internal boundaries that could mess with the spin of electrons and result in lost information. These boundaries – called domain walls – occur where the repeating pattern of atoms in the material doesn’t quite match up.
Recently, Tybell and his colleagues have found a way to make thin films from antiferromagnetic materials that look like they have no domain walls at all. By changing the arrangement of atoms – known as the lattice – in the substrate onto which the thin film is deposited, they can ensure the crystal grows in such a way as to avoid creating those internal boundaries. “Our key to controlling the physical properties is the lattice,” says Tybell.
“This is just a grey, boring image but actually what’s important is that each pixel has the same magnetic axis,” he says. “Suddenly you have no domain walls.”
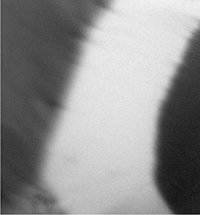
different magnetic regions, having
their ‘compass needle’ pointing in
different directions. The present
study shows how to engineer
materials with only one magnetic
ordering. Data taken in collaboration
with Ingrid Hallsteinsen, Rajesh
Chopdekar (SLS), Erik Folven and
Jostein Grepstad. Ref. I. Hallsteinsen,
E.Folven, F. Olsen, R.V.Chopdekar,
M.S. Rzchowski, C.B. Eom, J.K. Grepstad
and T. Tybell, APL Matr. 3, 062501 (2015);
licensed under a Creative Commons
Attribution (CC BY) license.
In recent years other researchers have shown that it’s possible to create a single crystal that doesn’t have domain walls. This new work shows it is possible in thin films, too. “That’s important because suddenly you have unprecedented new possibilities for devices,” says Tybell. “If you want to make a device that works you can’t work on a single crystal, you have to make thin films.”
There are still a number of challenges to overcome before spintronics goes mainstream, and Tybell says he can’t be sure how long it will be until you’ll be able to hold an entirely spintronic computer in your hand. “It will depend on how well we can control the materials to allow them to be mass produced,” he says. “I hope it’s soon, but I fear it’s quite in the future.”
But, he points out, the concept behind the transistors that are present in every computer around the world was first patented in 1925. It then took two decades until the first working transistor was realised by researchers working Bell Labs in the US, and several more years until they were in widespread use.
In the meantime, the materials Tybell and his colleagues are developing will not go to waste: they can also be used by researchers studying quantum objects from a fundamental physics point of view. And while it’s not easy to predict where that could lead, there’s always a chance it might prove vital in the future, one way or another.
“We should not forget that if you can grow single crystalline thin films, it opens up new avenues to study quantum phenomena and learn about these materials in a way that might be important for quantum technologies in the future,” he says. “I am sure there are many things still to discover.”
Kelly Oakes, February 2021
A list of NTNU papers related to nanoscience, nanotechnology and functional materials published in February 2021 may now found on NTNU Nano's page for publications.
This week's news articles describe the story behind the development of NTNU’s COVID-19 test, the new Norwegian Quantum Computing Centre, and how advances in silicon processing may lead to more environmentally friendly solar cells .
A list of NTNU papers related to nanoscience, nanotechnology and functional materials published in January 2021 may now found on NTNU Nano's page for publications.
Sometimes, when it comes to friction, less is more – at least that’s what several experiments over the last decade seem to have shown in the case of friction caused by layered materials. But it wasn’t until recently that researchers at NTNU figured out what was actually going on.
Friction probably isn’t something you think about on a daily basis, but as anyone who’s ever slipped over on an icy pavement could tell you, it can play a crucial role in many situations. The downside of friction, though, extends far beyond icy pavements: along with wear, it is responsible for approximately 23% of the world’s energy consumption.
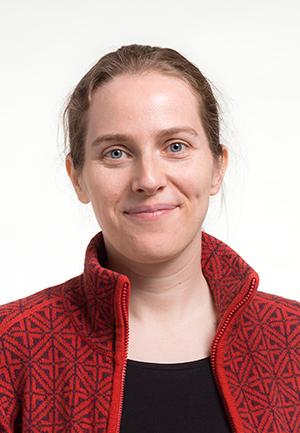
Photo: Thor Nielsen/NTNU
“Friction is a huge technological problem,” says Astrid de Wijn, a professor in the department of mechanical and industrial engineering at NTNU. “In industrialised societies, where we have machines that are moving constantly or very fast, friction is enormously costly.”
Studying friction is not as simple as classroom physics demonstrations involving a wooden block on a ramp suggest. “What is really happening is that the surfaces are rough and they meet at some points that are typically quite small,” says de Wijn. “When we study friction we are thinking about these contact points and how they behave.”
In order to really understand what they can see happening at the real world macroscale, researchers need to be able to explain the complex behavior of the materials at the nanoscale. “Many different things are happening at different length and time scales, and it makes friction very interesting,” says de Wijn.
Layered materials – such as graphene, which is a single layer of carbon atoms arranged in a honeycomb pattern – generally have low friction. They are already used in lubricants, but learning more about how they work could enable us to make the world’s machinery run more smoothly and reduce our energy bill on a global scale.
But in the last decade, researchers studying how friction works in materials like graphene have found that a single layer actually creates more friction than several layers. “People didn't understand that, and for years they were struggling with it,” says de Wijn. “They did simulations and they reproduced this behaviour but couldn't figure out what was really happening.”
The problem was, while there were plenty of results apparently showing what was causing the material to behave like this in particular situations, the explanations proposed by different researchers seemed to contradict each other, and nothing stood out. “They all had good arguments and evidence that, in their system, it was their suggested mechanism that was doing it, says de Wijn.
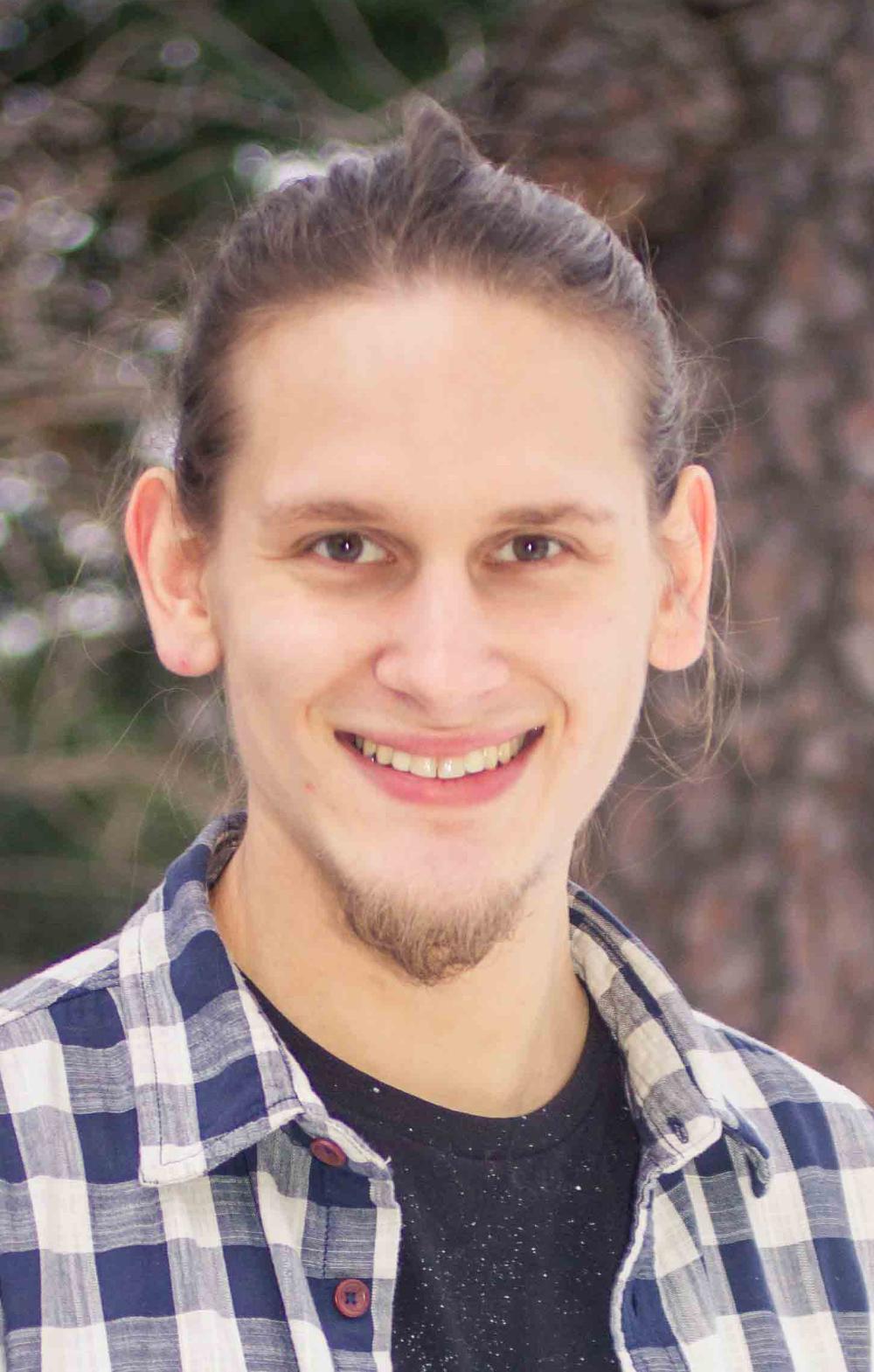
Photo: Marcus Gidekull
In a recent paper published in Nature Communications, de Wijn and PhD student David Andersson solved the problem. It turns out that all of the proposed solutions are, in a way, right.
One existing model that often proves helpful for understanding friction was first proposed in 1928 and consists of three elements: a support, a spring and a tip. The friction is then the force required to pull the tip across a sheet. While that works well for explaining many situations, it falls apart when layered materials are involved. So de Wijn and Andersson added just one variable to describe what is happening inside the layers of the sheet that the tip is being pulled across. “We didn't specify what that variable meant exactly – if it was a scrunching up of some kind, or some bending or one of the many possible things that people had proposed,” she says.
That simple tweak turned out to be the key to explaining several previous results, both from real world experiments and computational models. “Suddenly all the pieces fell into place and we understood what was happening,” says de Wijn. “It could be different mechanisms giving rise to the same kind of dynamics.”
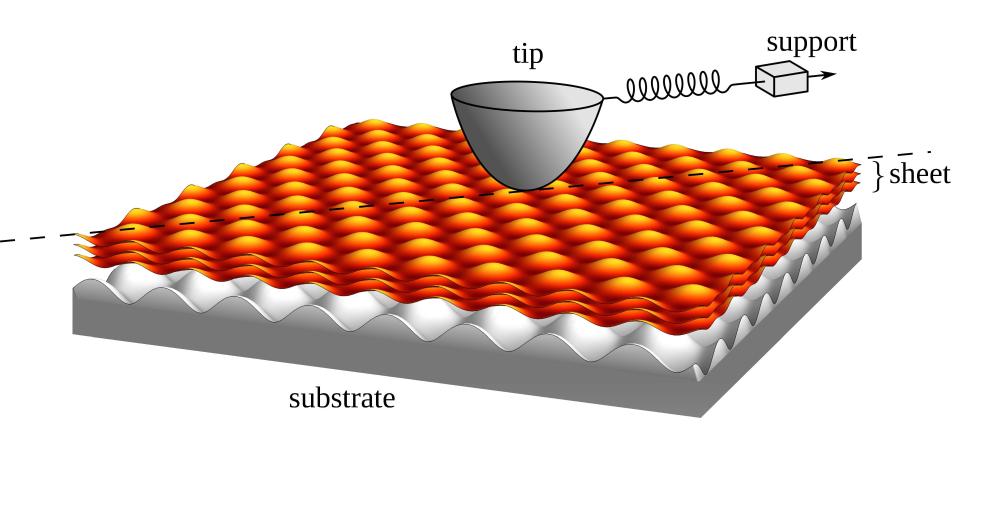
Unfortunately, because of travel restrictions due to the coronavirus pandemic, de Wijn and Andersson have not been able to present the work at many conferences or discuss it with colleagues as widely as they would otherwise have done – at least not in person. Nevertheless, now this puzzle has finally been solved, it opens up new avenues for investigating how friction works in layered materials, and could pave the way for new technology to reduce it.
The work is not over yet, though. The next step for de Wijn is to figure out how thermal fluctuations affect the system. “It's not just an academic puzzle for us,” she says. “Solving this means that we are one step closer to making friction lower.”
Kelly Oaks, January 2021
Upcoming events
Time: 14.15 - 15.45, December 11, 2023
Place: Lecture hall R9, the Natural Science Building
14.00 Coffee
14.15 Alois Herkommer: "Submicron 3D Printing: New Opportunities Through Two-Photon Polymerization"
14:50 Refreshments
15.10 Vegard Stubberud: “Experiences with two-photon printing at NTNU.”
15.30 End
The lectures are open to anyone and participation is free.
However, we kindly ask you to help us dimension the refreshments and food by registering here: NTNU Nano Lecture series
Study program
5 year study program in nanotechnology at NTNU (only in Norwegian)
Timini: Association for masterstudents in Nanotechnology (only in Norwegian)